The Double Helix: A Structural Marvel and Its Implications
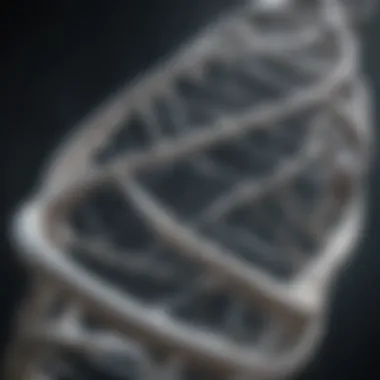
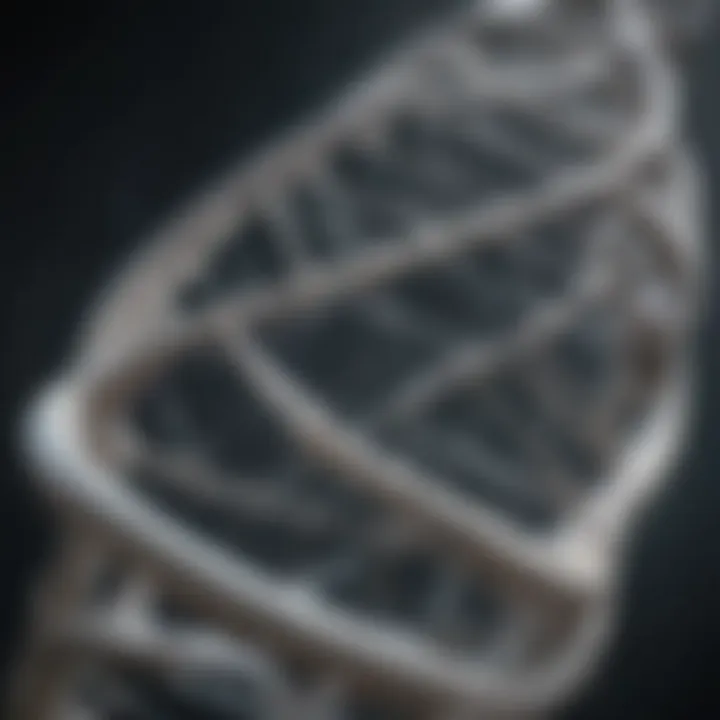
Intro
The double helix structure of DNA is more than just a scientific curiosity; it's the cornerstone of molecular biology and a crucial component in understanding life itself. Picture it: two intertwined strands, like a twisted ladder, each rung made of nitrogenous bases that dictate the genetic code. The discovery of this structure not only transformed biology but also propelled advances in genetics, biotechnology, and various scientific fields.
In this exploration, we peel back the layers of the double helix's significance, tracing its historical journey from initial discovery to its complex role in modern science. The narrative unfolds from the delicate dance of its molecular components to the robust implications that stretch into ethical questions in genetic engineering. As we venture into the labyrinth of knowledge that surrounds this fascinating structure, it reveals its role as a blueprint for life itself.
This structural marvel impacts far beyond the laboratory, weaving into the fabric of evolutionary theory and influencing the future of scientific inquiry. Each twist and turn of the double helix not only represents a building block of life but serves as a symbol of innovation and ethical dilemmas that will shape the future of humanity.
By the end of this article, we will gain a comprehensive understanding of not just DNA’s structure, but its profound implications across various fronts—scientific, ethical, and philosophical. This discussion promises to arm readers with insights into how this intricate architecture serves as a conduit for understanding the essence of biology and the potential it holds for the future.
Research Methodology
Description of Research Design and Approach
The investigation into the double helix begins by assessing the historical context and significance of its discovery, particularly the pivotal work of James Watson and Francis Crick in the early 1950s. Their research not only identified the structure but also laid the groundwork for future studies in genetics.
Materials and Methods Used in the Study
The article synthesizes a diverse array of scholarly sources, including original research papers, meta-analyses, and contemporary literature addressing genetic engineering and evolutionary theory. The methodology incorporates both qualitative and quantitative approaches, leveraging historical data to give depth to the ongoing discourse surrounding DNA. Critical thematic analysis helps in drawing connections between past discoveries and current implications in biotechnology and ethics.
Discussion and Interpretation
Interpretation of Results in Existing Literature
The implications of the double helix stretch far and wide. Genetic studies depend heavily on its structure. Research has demonstrated how variations in the DNA sequence lead to phenotypic diversity among organisms, contributing to evolutionary processes. Historical perspectives highlight both the excitement and fear surrounding genetic manipulation, particularly as we confront the nascent fields of CRISPR technology and personalized medicine.
"The nature of life can be understood through the lens of the double helix, illuminating pathways from our past to our uncertain future."
Implications for Future Research or Practical Applications
The promises and challenges posed by advances in genetic engineering are immense. For instance, while the ability to edit genomes holds potential for destroying genetic diseases, it also raises ethical questions that society must grapple with. Future research must tread carefully here, balancing the pursuit of knowledge with the responsibility to individuals and communities impacted by emerging technologies.
In summation, the double helix stands as a remarkable architectural feat of life, inciting continuous inquiry into its implications across disciplines. Understanding this structure will not only enlighten our grasp of biology but also guide future research in ethically sound practices that safeguard human interests.
Historical Perspective
The quest to understand the double helix structure of DNA is steeped in a rich historical tapestry that not only chronicles the progression of scientific thought but also underscores the collaborative nature of discovery in the realm of genetics. Understanding this historical fabric is essential, as it provides the context for the scientific breakthroughs that followed. By diving into the past, one gains insight into how early discoveries contributed to the monumental shift in biological sciences and laid the groundwork for modern genetics. Such an exploration highlights key elements such as the initial groundwork laid by pioneering researchers, the pivotal moments that sparked scientific interest, and the subsequent recognition of these achievements. This section aims to illuminate these historical landmarks and the impact they have had on current understandings of genetics and DNA.
Early Discoveries
Before Watson and Crick’s tenure, the enigma of hereditary material was in its infancy. Early 20th century researchers like Frederick Griffith and Oswald Avery opened the door to understanding that DNA was the carrier of genetic information. Griffith’s experiments in 1928 with pneumococcus bacteria suggested that a “transforming principle” could change harmless bacteria into virulent forms. This was further clarified by Avery’s 1944 work, where he demonstrated that DNA, not proteins, was responsible for this transformation. These findings dislodged long-held beliefs that proteins were the essence of heredity, steering the scientific community towards recognizing DNA's vital role.
Watson and Crick's Breakthrough
In 1953, James Watson and Francis Crick shocked the world with their description of the double helix structure of DNA. Utilizing models and prior research, including Rosalind Franklin’s X-ray diffraction images, they proposed a structure that elegantly linked the mechanisms of DNA replication to its overall function in biology. The model they unveiled depicted two intertwined strands, with complementary bases pairing in a manner that allowed for the exact copying of genetic material during cell division. This breakthrough was not just a scientific triumph; it was a conceptual leap that bridged molecular biology with the principles of heredity, reshaping how scientists viewed life itself.
Recognition and Nobel Prize
The scientific community recognized Watson, Crick, and Franklin's contributions in 1962 when they were awarded the Nobel Prize in Physiology or Medicine, along with Maurice Wilkins. This accolade marked a significant milestone not only for the researchers involved but also for the field of genetics as a whole. It signaled a shift in academic focus towards molecular mechanisms, ultimately paving the way for advancements in genetic engineering, biotechnology, and medical research. The legacy of this recognition has been profound, influencing countless studies and inspiring future generations of scientists to explore the intricate world of DNA and its functions.
"Understanding the history of the double helix allows us to appreciate not only the brilliance of past discoveries but also the limitless potential of future research."
Through this rich historical lens, one can see how the foundation laid by early geneticists contributed to our modern understanding of the double helix and its implications across various fields.
Structural Components of DNA
Understanding the structural components of DNA is central to grasping its role in genetics and molecular biology. At a fundamental level, DNA is not just a simple strand; it is a complex architecture comprised of various components that work together like a well-oiled machine. These components include nucleotides, the sugar-phosphate backbone, and the intricate base-pairing rules that ensure the stability and functionality of genetic material. Appreciating these elements is crucial because they provide insights into how genetic information is stored, replicated, and expressed.
Nucleotides: The Building Blocks
Nucleotides are the fundamental units that compose DNA, serving as the building blocks of this vital molecule. Each nucleotide consists of three parts: a nitrogenous base, a sugar molecule, and a phosphate group. The four types of nitrogenous bases are adenine, thymine, cytosine, and guanine. Each one contributes uniquely to the DNA structure, impacting the overall function and stability of the molecule.
Adenine
Adenine is one of the primary nitrogenous bases in DNA, playing a pivotal role in codifying genetic instructions. Its key characteristic is the formation of hydrogen bonds with thymine. This bond is one of the foundational elements of DNA replication and transcription. Adenine’s unique feature lies in its dual-ring structure, enabling it to interact well with complementary bases. This property enhances the stability of the DNA molecule. One advantage of adenine is that it pairs very specifically with thymine, leading to clearer genetic messaging; however, an overabundance can lead to mutations under certain conditions.
Thymine
Thymine is essential for the DNA molecule, particularly because it pairs with adenine in the structure. Importantly, thymine is distinguished by its unique methyl group attachment, providing stability to the DNA strand. This methylation means thymine can play a role in gene regulation, impacting gene expression levels. The pairing with adenine creates two hydrogen bonds, which are stronger than those formed by other bases. Thymine's key role, however, can be limited in certain organisms, where uracil takes its place in RNA, highlighting an evolutionary advantage in flexibility and function.
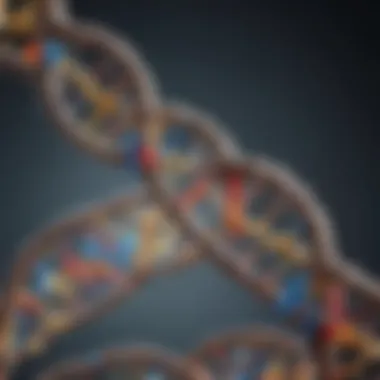
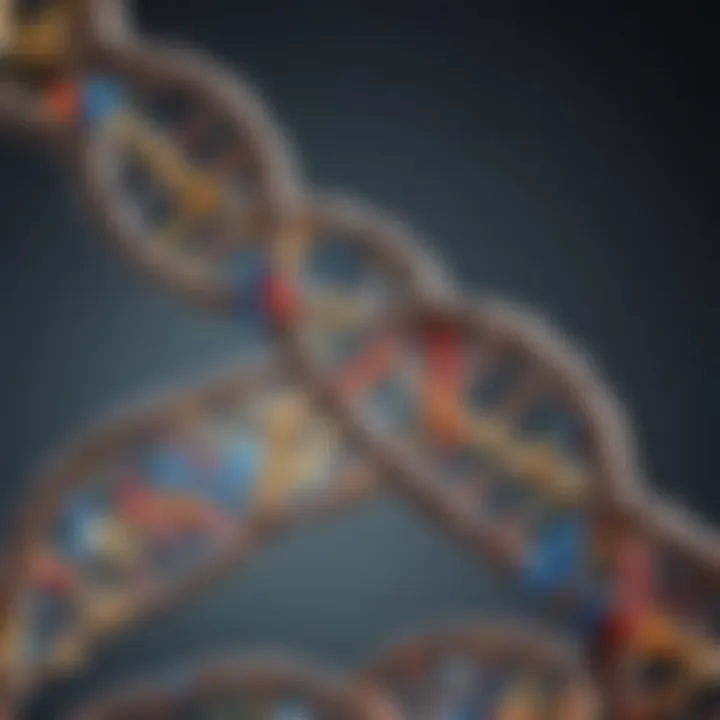
Cytosine
Cytosine is another nitrogenous base with distinct properties that impact genetic coding. It features a single ring structure, enabling it to bond with guanine via three hydrogen bonds. This strong connection contributes to the overall stability of the DNA double helix. Importantly, cytosine can undergo a biochemical change; in the presence of certain conditions, it can be converted into uracil. This adaptability highlights cytosine’s versatility in genetic processes, though it also presents a potential point of mutation. Such changes can lead to significant implications in genetic diseases and cellular functions.
Guanine
Guanine, like cytosine, features a double-ring structure and forms three hydrogen bonds with cytosine. This strong pairing is crucial, as it contributes significantly to the overall robustness of the DNA structure. One of guanine's unique features is its involvement in various metabolic pathways, including those involved in energy transfer and cellular signaling. This multifaceted role underscores its importance beyond simply being a part of the genetic code. However, its stability can be affected by certain chemical modifications during replication, which may bring about adverse effects in gene expression.
Sugar-Phosphate Backbone
The sugar-phosphate backbone functions as the structural framework of the DNA molecule. Composed of a repeating sugar and phosphate unit, it connects adjacent nucleotides, providing a stable yet flexible structure. This backbone's overall integrity is crucial for maintaining the DNA's double helix shape, which is essential for its biological functions. The alternating pattern of sugars and phosphates allows the genetic material to be both strong enough to endure cellular processes while also being adaptable for replication and transcription.
Base Pairing: The Complementarity Rule
The complementarity rule of base pairing ensures the accurate replication and expression of genetic information. This rule states that adenine pairs with thymine, while cytosine pairs with guanine. This specific pairing is not arbitrary; the hydrogen bonding patterns between these bases ensure that the DNA molecule remains stable yet accessible for cellular machinery. The proper pairing is vital for maintaining genetic fidelity during the replication process, reducing mutations, and ensuring that the inherited genetic information is propagated correctly.
Proper base pairing is fundamental to the integrity of DNA. If mismatches occur, it can lead to serious genetic consequences, including diseases and disorders.
Overall, these structural components not only underpin the physical characteristics of DNA but also play a significant role in its various functions within biological systems. Recognizing the intricacies of these components provides a clearer picture of how genetic information is managed and utilized in living organisms.
The Double Helix Structure
The structure of the double helix stands as a cornerstone of molecular biology, portraying not just design, but the very mechanics of life. It's not just about entwined strands of DNA; it’s a blueprint that underpins biological processes, a symbol of life's complexity captured in an elegantly simple form. Understanding this structure can unlock countless questions about genetics, evolutionary biology, and even biotechnology.
Geometry and Dimensions
At its heart, the geometrical arrangement of the double helix is surprising in its precision. Each full turn of the helix measures about 10 base pairs, just shy of three nanometers in diameter. This consistent structure ensures that the genetic material can be tightly packed within the confines of the cell nucleus without losing accessibility. This arrangement allows for efficient storage and retrieval of information essential for cell function and replication.
The helical twist itself, defined by a right-handed chirality, optimally exposes the bases—adenine, thymine, cytosine, and guanine—allowing for effective hydrogen bonding between complementary strands. This geometry isn’t arbitrary; it’s evolved to promote stability while simultaneously allowing for the flexibility necessary during replication and transcription.
Major and Minor Grooves
Another significant feature of the double helix is the presence of major and minor grooves, which are vital for protein interactions. These grooves provide distinct access points for regulatory proteins to bind to the DNA. It’s like having a lock with multiple keys—each groove can be viewed as a different entry point for various proteins that influence gene expression.
- Major Groove: Wider and more accessible to larger proteins, it often serves as the primary site for transcription factors and other DNA-binding proteins.
- Minor Groove: While narrower, this groove is still critical for interactions, especially for smaller molecules and certain types of medications, as they can fit snugly and regulate DNA functions.
Understanding these grooves is crucial, not only for comprehending how genes are turned on or off but also for developing drugs that can specifically target these sites, potentially paving the way for innovations in treatment.
Stability and Flexibility
The balance between stability and flexibility in the double helix is a remarkable illustration of nature's engineering prowess. The hydrogen bonds between the complementary bases ensure that the molecule remains stable under various conditions. However, this stability doesn't compromise its ability to allow essential cellular processes to take place.
For instance, during replication, the strands must separate, a task made possible not just through chemical energy but also due to the intrinsic flexibility of the DNA structure. This flexibility allows the double helix to undergo twists and turns, accommodating the needs of different proteins that interact with it during processes like replication and transcription.
In light of these factors, the double helix is more than a static structure—it's a dynamic entity essential for life on a molecular level. Its geometry, groove accessibility, and the delicate balance of stability and flexibility not only highlight the complexities of genetics but also invite further exploration into the mysteries of life's molecular foundation.
DNA Replication Process
Understanding DNA replication is like peeling back the layers of a complex onion; each layer reveals critical components of how genetic information is faithfully copied and transmitted from one generation to the next. This process is fundamental not just to the field of molecular biology, but also to genetics, cell biology, and even the future of gene therapy. The steps of DNA replication ensure that when a cell divides, each new cell inherits an exact copy of the DNA, which is vital for growth, development, and maintenance of all living organisms.
The importance of DNA replication can be summed up in a few key points:
- Accuracy: DNA replication must be precise to preserve genetic information.
- Speed: The process needs to be efficient enough to allow organisms to grow and reproduce in a timely manner.
- Regulation: Various checkpoints in the replication cycle can help prevent errors that might lead to mutations or diseases, such as cancer.
Initiation of Replication
The initiation of replication marks the beginning of this intricate process. Before anything else, the DNA must be unwound. Specific sites on the DNA known as origins of replication serve as starting points. The double helix at these points is separated by enzymes called helicases, which break the hydrogen bonds between the nitrogenous bases, effectively opening the helical structure like a zipper.
Next, the enzyme primase synthesizes a short RNA primer complementary to the DNA template. This primer serves as a launching pad for DNA synthesis, as DNA polymerase cannot start a new strand de novo. It's quite fascinating; without this first step, the entire replication process would grind to a halt.
A crucial detail sometimes overlooked is that replication occurs in a specific direction. The leading strand, which elongates towards the replication fork, contrasts with the lagging strand that elongates away from it, leading to the synthesis of short segments known as Okazaki fragments.
Elongation and Proofreading
Once initiation is complete, the elongation phase begins, with DNA polymerase working diligently to add nucleotides to the growing strand, following the rules of base pairing—adenine with thymine, and cytosine with guanine. The speed and efficiency of elongation are impressive, as it can occur at rates of about 1000 nucleotides per second in human cells.
However, errors can happen, and this is where proofreading comes into play. DNA polymerases have a built-in proofreading ability; they can recognize and correct mispaired bases. If an incorrect nucleotide is added, the enzyme has the capacity to backtrack, remove the wrong nucleotide, and replace it with the correct one. This remarkable quality vastly reduces the error rate, making DNA replication remarkably accurate.
Termination of Replication
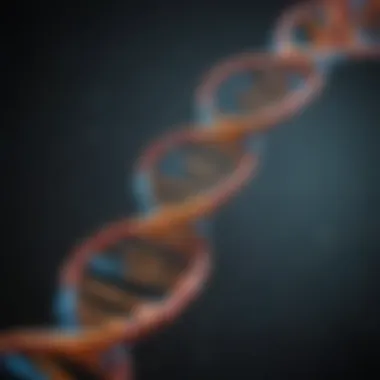
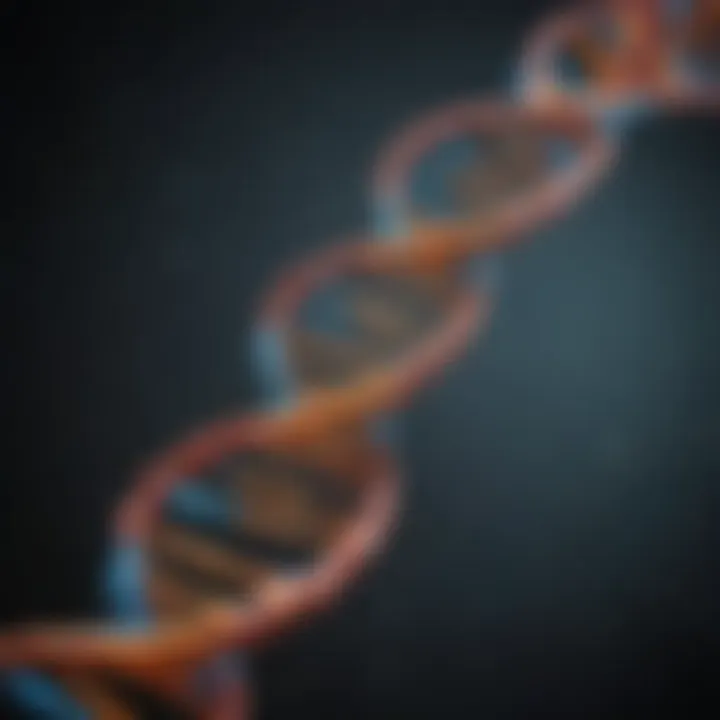
As the replication forks continue to move away from the origins, they eventually meet each other, leading to the termination phase. When the two forks converge, various proteins help in finalizing the newly synthesized strands. In prokaryotes, like bacteria, the circular DNA simply separates, while in eukaryotes, various mechanisms work to ensure that the linear DNA strands are fully complete and that all necessary proteins are in place.
A critical aspect of termination is the replacement of RNA primers with DNA. Special enzymes called exonucleases remove the RNA, allowing DNA polymerase to fill in those gaps with DNA nucleotides. Once this transition is complete, the final twist in the tale is that the DNA strands naturally re-form into their characteristic double helix structure.
"DNA replication is a showcase of nature’s engineering prowess, demonstrating how life replicates itself with remarkable accuracy."
For further exploration of these concepts, you can visit these resources:
Gene Expression and Regulation
Gene expression and regulation is a crucial theme within the framework of genetic science. It acts as a thermoregulator of the cellular machinery, controlling how genes dictate the synthesis of proteins and subsequent traits in organisms. The significance of understanding this process cannot be overstated, as it contributes to the broader narrative of how life operates at a molecular level, with implications stretching across multiple disciplines, including medicine, genetics, and biotechnology. The spin of a double helix isn’t just a captivating shape but a blueprint guiding everything from cellular development to evolutionary adaptation.
Transcription Mechanism
The transcription mechanism symbolizes the first pivotal step in gene expression, where specific sections of DNA are transcribed into messenger RNA (mRNA). This process can be segmented into several noteworthy phases. Initially, an enzyme called RNA polymerase binds to the promoter region of a gene. Once attached, it unwinds and separates the DNA strands, allowing the synthesis of a complementary RNA strand. The phenomenon is somewhat like a chef following a recipe from a well-thumbed cook book; the original recipe stays intact, while a new dish is prepared.
This mRNA strand, once processed and spliced to remove non-coding sequences, migrates to the cytoplasm, where it serves as a template for the next step—translation. It's essential to highlight that this process can be influenced by various factors, including transcription factors, which can either enhance or suppress gene expression.
Translation Process
With mRNA in tow, the next stage is translation, where the genetic code is interpreted to synthesize proteins. This occurs in the ribosomes, often referred to as the cellular assembly lines. The mRNA sequence is read in sets of three nucleotides, known as codons, each coding for a certain amino acid. The process involves transfer RNA (tRNA), which acts like a delivery truck, bringing amino acids to the ribosome, matching them according to the mRNA sequence.
- Initiation: The start codon is recognized, and the ribosome assembles with the mRNA and the first tRNA.
- Elongation: tRNAs continue to add amino acids to the growing polypeptide chain, following the sequence dictated by the mRNA.
- Termination: When a stop codon is reached, the assembly disbands, releasing the newly formed protein.
This protein then undergoes modifications and folding, becoming functional in guiding various cellular processes. Each translation round is not only a testament to genetic coding but also a marvel of precision and efficiency.
Regulatory Elements
Regulation of gene expression ensures that proteins are produced in the right cells, at the right times, and in appropriate amounts. It’s as if a conductor is shaping the symphony of molecular biology, ensuring harmony among various parts. Regulatory elements play an integral role here, consisting of enhancers, silencers, and promoters.
- Enhancers can boost transcription levels and can act over long distances along the DNA chain.
- Silencers, conversely, can inhibit gene expression, demonstrating the balance necessary for proper cellular function.
- Promoters are essential for the initiation of transcription, acting as landing pads for RNA polymerase.
Further complexity arises from epigenetic modifications; these changes can influence gene expression without altering the DNA sequence. Methylation and histone modifications present ways in which environmental signals can dictate gene activity, demonstrating adaptability of organisms.
"Gene expression is the bridge that connects the genotype of an organism with its phenotype, driving diversity and adaptability among species."
Applications of Double Helix Knowledge
Understanding the double helix structure of DNA has opened the floodgates to numerous applications in diverse scientific fields. It’s difficult to overstate just how significant this understanding is, as it underpins advances not only in genetics but also in biotechnology and medical sciences. Each application harnesses the chandelier of knowledge derived from the double helix, influencing modern science and industry in ways that were once the stuff of science fiction.
Genetic Engineering
Genetic engineering stands as a prime example of how double helix knowledge transforms practical applications. At its core, genetic engineering involves modifying the genetic makeup of organisms. This can be anything from altering the genes of crops to make them resistant to pests to creating genetically modified organisms (GMOs) that can produce medicines or clean pollutants. Such precise manipulation offers a buffet of benefits:
- Increased agricultural yields
- Enhanced nutritional content of food
- Development of disease-resistant plants and animals
Furthermore, the accessibility to gene-editing tools, such as CRISPR-Cas9, owes much to the understanding of the DNA structure. Researchers can now act much like skilled artisans, snipping and splicing the genetic code at desired locations, customizing it to satisfy essential requirements. This ability to modify genes at such a granular level is like wielding a fine-tipped brush on a canvas.
Biotechnology Innovations
The marriage of double helix insights and biotechnology has birthed revolutionary innovations. Biotechnology refers to the use of biological systems or living organisms to develop products. One cannot ignore how the structure of DNA infuses life into biotechnology. Consider the development of biopharmaceuticals—drugs created from biological sources.
Here are a few remarkable advancements:
- Insulin production: Recombinant DNA technology has enabled the production of insulin. This is a game-changer for diabetic patients, as it allows for the mass production of insulin that is biologically identical to human insulin.
- Gene therapy: Innovations in gene therapy are realigning approaches to treat genetic disorders. By correcting defective genes, there is a potential for cures rather than just management.
- Synthetic biology: This nascent field crafts new biological parts, devices, and systems. It parses the double helix into bits and pieces, constructing new functions or capabilities.
Medical Advancements
Health care’s landscape has dramatically shifted due to insights gleaned from the double helix. Medical advancements connect various dots between genetics and disease management. With the surge in understanding genetic predispositions to certain illnesses, the focus often lies on personalized medicine.
This approach allows:
- Tailoring treatments based on an individual’s genetic make-up, ensuring efficacy and minimizing side effects.
- Unlocking potential cures for rare genetic disorders through targeted therapies.
- Screening techniques that can identify predispositions to diseases long before symptoms surface—effectively allowing for early interventions.
The double helix is more than a structure; it’s a blueprint that caters to the intricacies of life itself.
Double Helix and Evolution
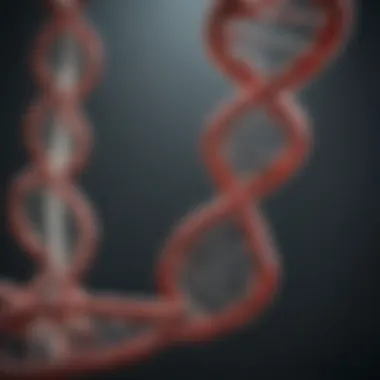
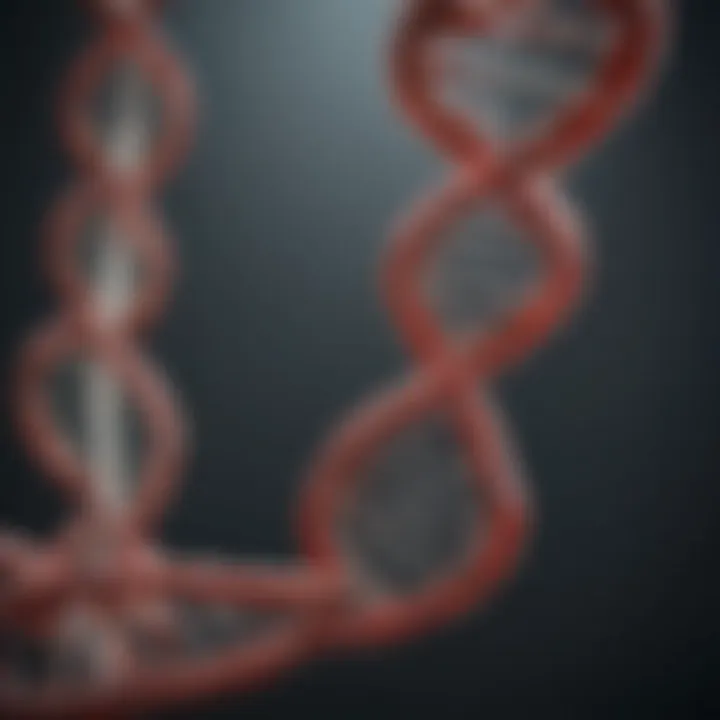
The relationship between the double helix structure of DNA and the theory of evolution is a compelling focal point in understanding how life has developed on Earth. The structure serves as a fundamental blueprint that encodes genetic information, enabling the process of heredity and natural selection. By dissecting the interplay between these two concepts, we gain insights into the mechanisms that drive biological diversity and adaptation over time.
Molecular Evidence of Evolution
Molecular biology provides significant evidence supporting evolutionary theory, much of which stems from the understanding of DNA structure. The double helix aids in delineating how genetic mutations, which are occasional changes in the DNA sequence, contribute to evolutionary changes. For instance, when random errors occur during DNA replication, they can lead to variations in organisms. These variations might be beneficial, neutral, or harmful. Numerous examples document this phenomenon.
- Homologous Genes: Genes found in different species that share a common ancestry often exhibit similarities. For example, the genetic sequences of humans and chimpanzees are remarkably similar, supporting the idea of a common ancestor.
- Vestigial Structures: The genetic coding for traits that serve diminished or no function in contemporary organisms, like the wings in flightless birds or the pelvic bones in whales, also traces back to their evolutionary origins, partially illuminated through DNA analysis.
"The study of molecular evolution allows scientists to track the nuances of genetics across generations, forming a clearer picture of life’s journey."
Beyond species comparison, DNA evidence can also shed light on evolutionary timelines. Molecular clock techniques allow us to estimate the time of divergence between species based on the rate of genetic mutations, showcasing the temporal dimension of evolution.
Phylogenetic Analysis
Phylogenetic analysis, the study of the evolutionary relationships among biological entities, leverages DNA sequencing to construct family trees, elucidating how species have diverged from common ancestors over millions of years. This technique bares the utility of the double helix structure, as it is the very foundation upon which genetic similarities and differences are evaluated.
- DNA Barcoding: This method uses a short genetic marker in an organism’s DNA to identify it. This information can highlight evolutionary relationships among various species, aiding in conservation efforts or classifying biodiversity.
- Comparative Genomics: It examines the similarities and differences in the DNA sequences of different species, revealing evolutionary patterns. By comparing entire genomes, researchers can pinpoint genetic adaptations that have occurred in response to environmental pressures.
In essence, phylogenetic analysis not only confirms the interconnectedness of life but also emphasizes the double helix's pivotal role as the carrier of evolutionary information. The ability to use molecular data effectively illustrates how complex traits have emerged and adapted over time, ultimately framing our understanding of biodiversity today.
Ethical Considerations in Genetics
The advancement of genetics, especially with the scaffold of the double helix' structure, has opened a whole can of worms regarding ethics. Understanding these implications isn't just for scientists; it’s crucial for everyone, whether you're a student, educator, or a professional in the field. As we analyze how our grasp of genetics can shape society, we need to tread lightly on issues that not only affect individual lives but humanity as a whole.
In retrospect, the capacity to edit genes or manipulate DNA might seem like a miracle of modern science. These modifications present potential adventures in therapeutic treatments for genetic disorders, but one can't help but wonder where we draw the line. The ability to alter life—a power previously reserved for evolution—is now in human hands, commanding us to consider the underlying moralities involved.
"With great power comes great responsibility."
This adage might ring true here, echoing the sentiment that every step we take in genetic modification should be accompanied by thoughtful deliberation of potential consequences. What if genetic engineering becomes a tool for privilege? Will wealth and status dominate access to the benefits of such technology? The implications of creating a class of 'designer babies' highlight this concern. The fear is not just about the ease of genetic customization; it's about the resulting divide in society, solely based on abilities to afford these enhancements.
Moreover, genetic modification ethics can't escape from questions about consent. If genetic alterations are made at the embryonic stage, how can future individuals express their stance on such changes? Unquestionably, this appears to infringe upon their autonomy.
Lastly, as we engage with these substantial modifications, we must ponder the ecological balance. Every act of modification impacts complex webs of relationships within ecosystems. Every alteration can ripple out and affect other organisms, leading to potential ecological disruptions.
Key Considerations for Genetic Modification Ethics:
- Access Inequality: Who gets to modify and who doesn’t, potentially leading to a genetic elite?
- Consent: How do we approach consent when altering future generations?
- Ecological Impact: What effects may arise in the surrounding ecosystems from modified organisms?
In all, ethics in genetic modification urges a thorough inquiry and persistent dialogue, necessitating engagement from all facets of society as we navigate this new frontier.
Privacy Concerns in Genetic Data
With the burgeoning field of genetics, privacy concerns in genetic data command our attention. Each DNA sequence tells an intricate tale unique to every individual, containing sensitive and personal information. As we strive for progress, safeguarding this data is paramount.
There is an ongoing tussle between the benefits of genetic research and the rights to personal privacy. Genetic data has the potential to unveil a treasure trove of information, not just on the individual but also their relatives. This intertwined nature raises a pressing question: Who owns this genetic information?
The fear of misuse of data lurks in every corner—whether it’s for discriminative purposes (such as employment or insurance biases) or for ghosting an individual’s genetic predispositions. As genetic information is increasingly collected, the risk of breaches in data security becomes a reality. A hacker gaining access to a repository of genetic data can unleash devastating consequences, potentially impacting numerous personal lives.
Moreover, regulations in place regarding genetic data often seem to lag significantly behind the pace of advancements. Although some guidelines exist, they need constant reevaluation against emerging technologies and methods of data handling. If we don't evolve with these advancements, we risk a scenario where personal identities become fodder for corporate profit.
Key Privacy Considerations in Genetic Data:
- Data Ownership: Navigating who has rights to genetic data.
- Risk of Discrimination: Addressing how genetic information can be weaponized against individuals.
- Data Security: Ensuring robust measures are in place to prevent breaches.
Future Research Directions
The path forward in genetic research brims with potential, driven by the double helix's structural elegance and functional significance. As scientists continue to decipher the secrets wrapped within this helical framework, future research directions promise to broaden our understanding of genetics and its many applications. This exploration holds vital implications for medicine, agriculture, and various other fields, making the inquiry into these directions extraordinarily relevant.
CRISPR and Gene Editing Technologies
One of the most exciting avenues is the advancement of CRISPR technology. This gene-editing tool, inspired by the natural defense mechanisms of bacteria, allows researchers to make precise modifications to DNA sequences. The crux of CRISPR's power lies in its simplicity and efficiency, enabling alterations that were time-consuming or inaccurate with previous methods.
- Precision: Unlike traditional genetic modification techniques, CRISPR targets specific DNA locations with impeccable accuracy. This minimizes off-target effects, making it a preferred choice for researchers.
- Accessibility: The instructional nature of CRISPR is attractive for laboratories lacking extensive funding. Essential reagents can be made in-house, broadening the reach of genetic research to institutions in diverse socioeconomic contexts.
- Applications: The myriad applications of CRISPR are astounding. From potential cures for genetic disorders like sickle cell anemia to modifications that might enhance crop resilience against diseases, the implications of this technology are immense.
"The age of gene editing is upon us, and CRISPR stands at the forefront, ushering a revolution in how we perceive genetics and its applications."
Yet, the ethical considerations surrounding CRISPR demand scrutiny. Discussions on its appropriateness for germline editing often spark heated debates within scientific and public communities. Balancing innovation with ethical responsibility becomes crucial as this technology evolves.
Synthetic Biology Prospects
Synthetic biology, another significant direction, involves redesigning organisms for beneficial purposes. Here, the principles of engineering are applied to biology, helping create novel systems that can perform tasks previously deemed unattainable. The integration of synthetic biology with our understanding of the double helix brings about several possibilities:
- Designing Minimal Cells: Efforts to construct minimal versions of cells can teach us about essential biological functions. This knowledge can lead to solutions in metabolic engineering and the production of biochemicals.
- Bioproduction: By engineering microbes to produce complex molecules, synthetic biology offers sustainable alternatives in pharmaceuticals, biofuels, and other industrial products. Imagine scoring high yields of valuable compounds from engineered bacteria instead of conventional extraction methods.
- Environmental Solutions: Synthetic organisms could potentially remediate polluted environments or capture carbon dioxide, contributing to sustainability efforts. Researchers are exploring pathways to design organisms that can thrive in extreme conditions, opening doors to biotechnological applications in various environments.
Continued research in synthetic biology not only expands our understanding of genetic material but also allows for practical applications that positively impact society. As we forge ahead, it becomes essential to navigate the landscape of synthetic organisms with ethical foresight and regulatory mindfulness.
With the double helix serving as both a foundation and inspiration, the inquiries into CRISPR technology and synthetic biology mark a new frontier in genetics, positioning us to address pressing challenges while contemplating the future of life itself.