A Comprehensive Guide to DNA Replication Mechanisms
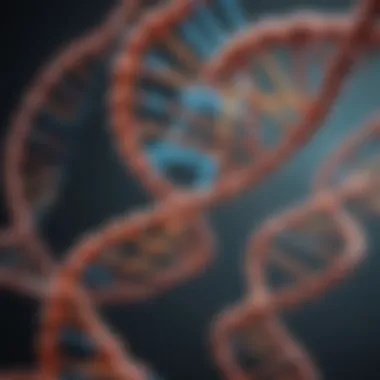
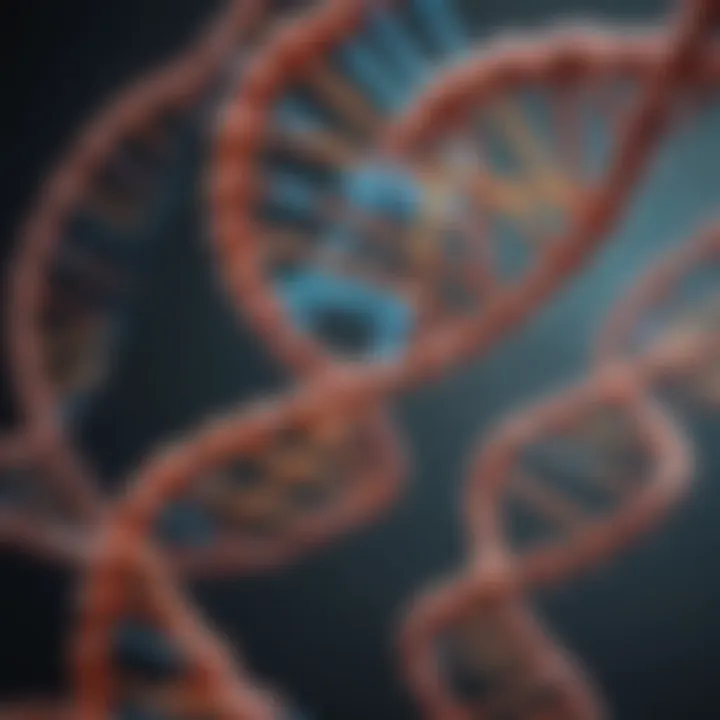
Intro
DNA replication is a vital process that ensures the accurate duplication of genetic information. This process is essential for cellular division and reproduction, impacting countless biological functions. Understanding DNA replication involves delving into its intricate mechanisms, the roles of specific enzymes, and its broader significance in genetic fidelity and evolution.
The complexity of DNA replication lies in the coordination of multiple proteins and enzymes that interact at various stages. In this guide, we examine the distinct phases of replication, highlighting the contributions of key players such as DNA polymerases and helicases. Furthermore, the implications of recent research provide additional insight into the dynamic nature of replication and its significance in fields such as genetics, molecular biology, and medicine.
"Understanding DNA replication not only sheds light on fundamental biological processes but also opens doors for advancements in therapeutic techniques and genetic engineering."
As we navigate through the various components of DNA replication, we will uncover the research methodologies that underpin our current understanding, interpret findings in the context of existing literature, and discuss the potential for future inquiries and applications.
Prologue to DNA Replication
DNA replication is a critical biological process that ensures genetic material is faithfully copied before cell division. Understanding the nuances of this process is foundational in molecular biology, genetics, and biotechnology. This section discusses the essential concepts and underlying significance of DNA replication, which forms the core of cell function and perpetuation.
Fundamental Concepts of DNA
DNA, or deoxyribonucleic acid, is the molecule that carries the genetic instructions for life. Its structure is often described as a double helix, made up of nucleotides, each containing a phosphate group, a sugar, and a nitrogenous base. There are four types of nucleotides in DNA: adenine, thymine, cytosine, and guanine. The sequence of these bases encodes genetic information, which guides cellular activities and heredity.
These fundamental concepts set the stage for understanding DNA replication. During replication, the two strands of the double helix separate, allowing each strand to serve as a template for synthesizing a new complementary strand. This semi-conservative mechanism ensures that each new DNA molecule contains one original strand and one newly synthesized strand. This method is crucial for preserving genetic fidelity across generations.
Importance of DNA Replication
The importance of DNA replication cannot be overstated. It is the mechanism that enables organisms to grow, reproduce, and repair damaged cells. Without accurate DNA replication, cells would not be able to maintain their integrity, leading to mutations or cell death.
"Accurate DNA replication is vital for life. It prevents errors that can lead to diseases such as cancer."
Moreover, advancements in understanding DNA replication have transformed various fields such as genetics, molecular biology, and medicine. For instance, the knowledge gained about DNA replication has paved the way for techniques like the Polymerase Chain Reaction (PCR), which allows for the amplification of specific DNA sequences. This has impactful applications in medical diagnostics, forensic science, and genetic research.
In summary, DNA replication is a complex yet essential process that plays a pivotal role in sustaining life. Grasping its fundamental principles and significance lays the groundwork for exploring the mechanics of DNA replication further.
The Structure of DNA
Understanding the structure of DNA is crucial for grasping how genetic information is stored, replicated, and expressed. DNA, or deoxyribonucleic acid, serves as the blueprint for life. Its intricate structure facilitates not only the storage of genetic information but also its transfer and use across generations. Key elements of DNA's structure contribute to its stability and functionality, making it a central focus in molecular biology and genetics.
Double Helix Formation
The double helix is the iconic structure of DNA, discovered by James Watson and Francis Crick in 1953. This formation consists of two long strands of nucleotides intertwined, resembling a twisted ladder. Each strand is composed of repeating units of nucleotides, which include a phosphate group, a sugar molecule, and a nitrogenous base. The two strands are oriented in opposite directions, described as anti-parallel. This orientation is critical for the processes of replication and transcription.
The nitrogenous bases pair in specific combinations—adenine pairs with thymine, and cytosine pairs with guanine—through hydrogen bonds. This complementary base pairing is key to the accuracy of DNA replication, ensuring that the genetic code is preserved in daughter cells. The stability provided by the double helix structure also protects the genetic material from damage.
Nucleotide Composition
DNA is composed of four types of nucleotides, each differing by their nitrogenous base. These bases are adenine (A), thymine (T), cytosine (C), and guanine (G). The sequence of these bases encodes the genetic information that directs the development and functioning of living organisms.
- Adenine: A purine that pairs with thymine.
- Thymine: A pyrimidine that pairs with adenine.
- Cytosine: A pyrimidine that pairs with guanine.
- Guanine: A purine that pairs with cytosine.
The sugar in the nucleotide structure is deoxyribose, which distinguishes DNA from RNA (ribonucleic acid). The phosphate groups link the sugar molecules together, forming the backbone of the DNA strand. This sequence of nucleotides determines the unique genetic code of an organism and is essential for processes such as replication and transcription.
"The sequence of nucleotides in DNA is fundamental to the diversity of life – it is the language of biology."
The precise arrangement of these nucleotides is what allows DNA to carry out its various functions, from encoding proteins to regulating cellular activities. Thus, the structure of DNA not only provides insight into its roles but also lays the groundwork for our understanding of genetics and biotechnology.
Mechanisms of DNA Replication
DNA replication is a fundamental process ensuring the accurate copying of genetic material. Understanding the mechanisms underlying this process is crucial for appreciating how cells maintain genetic integrity. The mechanisms not only involve a finely-tuned orchestration of enzymes but also regulatory factors that ensure the fidelity and timing of replication.
Semi-Conservative Replication
The concept of semi-conservative replication is critical to our understanding of DNA replication. Proposed by Watson and Crick in the early discoveries of DNA structure, this method entails the separation of the two strands of the DNA double helix. Each strand serves as a template for synthesizing a new complementary strand.
In semi-conservative replication, the result is two DNA molecules, each containing one original strand and one newly synthesized strand. This process significantly reduces errors in copying, as each new DNA molecule retains half of the original information. The primary benefit here is error correction, which is vital for the survival of an organism.
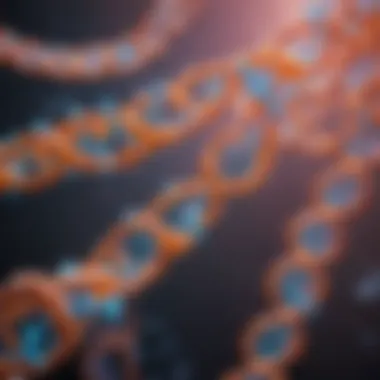
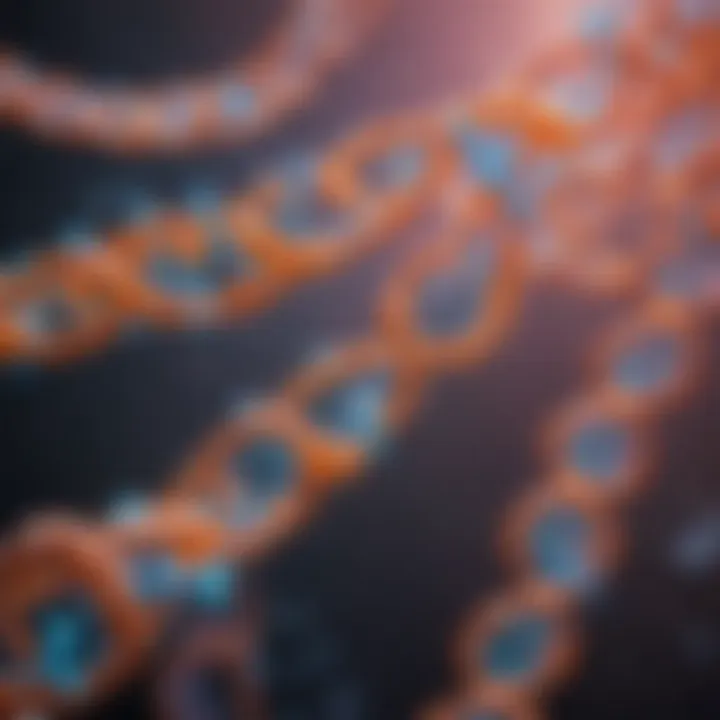
- Initiation: The unwinding of DNA begins at specific locations known as origins of replication. Enzymes like DNA helicase open up the double helix.
- Template Strands: Each strand acts as a template, guiding the addition of complementary nucleotides by DNA polymerase.
- Product Formation: Ultimately, two identical DNA helices emerge, conserving genetic information.
This method not only emphasizes the accuracy of DNA replication but also illustrates the dynamics between preservation and variation in genetic material.
***"
!> "Understanding semi-conservative replication formed the basis of molecular genetics, illustrating how life preserves its complexity across generations."
Replication Fork Dynamics
The replication fork is a pivotal structure during DNA replication, representing the Y-shaped region where the double helix separates. The dynamics at the replication fork are vital for the continuity and speed of DNA synthesis.
As the replication fork moves along the DNA, several key events transpire. First, the helicase continues to unwind the DNA strands while single-strand binding proteins stabilize the unwound sections, preventing them from re-annealing.
Moreover, the leading strand is synthesized continuously, whereas the lagging strand is synthesized in short fragments known as Okazaki fragments, later joined together by DNA ligase. This disparity in synthesis rates underscores the complexity of the process.
Factors to consider about replication fork dynamics:
- Speed of Replication: The rate at which the fork advances can influence cellular division and overall genetic stability.
- Stability: Various proteins help manage the structure, keeping the fork resilient against mechanical stress and replication errors.
- Checkpoint Signaling: Cells assess the progress at the replication fork, allowing for repair mechanisms to act if abnormalities arise.
Key Enzymes Involved in Replication
The process of DNA replication is an essential activity that preserves genetic information across generations. Key enzymes play a crucial role in this intricate process. Their specificity and function ensure that DNA replication is accurate and efficient, maintaining the fidelity of genetic material. Understanding these enzymes provides insight into the broader implications of DNA replication for cellular function.
DNA Helicase
DNA helicase is an essential enzyme that initiates the process of DNA replication. It unwinds the double helix structure of DNA, separating the two strands and creating the replication fork. This unwinding is necessary for access to the genetic code by other enzymes involved in replication.
The activity of DNA helicase involves ATP hydrolysis, where energy derived from ATP is used to break the hydrogen bonds between nucleotide pairs. This action not only opens the strands but also prevents them from re-annealing.
"DNA helicase is crucial because if the strands do not separate efficiently, replication can be stalled, leading to errors that might cause mutations."
DNA Polymerase
DNA polymerase is perhaps the most studied enzyme involved in DNA replication. It synthesizes new DNA strands by adding nucleotides complementary to the template strand. This enzyme operates primarily in three phases: initiation, elongation, and proof-reading.
In the initiation phase, DNA polymerase requires a primer, which is a short single-stranded RNA segment synthesized by primase. Once the primer is in place, DNA polymerase begins the elongation process, adding nucleotides to the growing strand in the 5' to 3' direction. This function is vital for the overall accuracy of DNA replication.
Moreover, DNA polymerase has an intrinsic ability to proofread newly synthesized DNA. This means it can detect and correct mismatches in base pairing, which further ensures the fidelity of DNA replication.
Ligase and Primase
Ligase and primase are additional enzymes that, while less discussed, are critical to the replication process. Ligase is responsible for joining Okazaki fragments on the lagging strand. During replication, the lagging strand is synthesized in small pieces, and ligase connects these fragments, sealing nicks between adjacent sections of DNA. This step is crucial for maintaining the integrity of the newly synthesized strand.
Primase, on the other hand, synthesizes short RNA primers necessary for DNA polymerase to begin its work. Without primase, DNA polymerase would not have a starting point for DNA synthesis. The timing and regulation of these enzymes ensure a smooth and effective replication process.
In summary, the key enzymes involved in DNA replication work in harmony to create accurate copies of genetic material. Their functions are indispensable for cellular reproduction, and any errors in their activity can lead to serious consequences for cellular function and organismal health.
Stages of DNA Replication
DNA replication is a fundamental biological process. It is essential for the accurate duplication of genetic material during cell division. Understanding the stages of DNA replication is vital because it helps to grasp how life perpetuates itself at the molecular level. This portion of the article will delve into the three key stages: initiation, elongation, and termination. Each stage has its own set of processes and significance contributing to the overall fidelity of DNA replication.
Initiation
Initiation is the first stage of DNA replication. This process begins at specific locations on the DNA molecule called origins of replication. The recognition of these sites is facilitated by several proteins that bind to the origin, forming a complex known as the pre-replication complex. Within this phase, DNA helicase plays a crucial role. It unwinds the double helix structure of DNA, creating two single-stranded templates. The separation of strands is critical because it allows enzymes access to the individual strands for synthesis.
Once unwound, a short RNA primer is synthesized by the enzyme primase. This primer provides a starting point for DNA synthesis, since DNA polymerases cannot initiate synthesis without a primer. The result is two templates ready for replication, marking the transition to the elongation phase.
Elongation
Elongation is where the main work of DNA replication occurs. During this stage, DNA polymerase enzymes add nucleotides to the growing DNA strand, complementary to the template strand. This process occurs in a 5' to 3' direction, meaning new nucleotides are added to the 3' end of the growing chain.
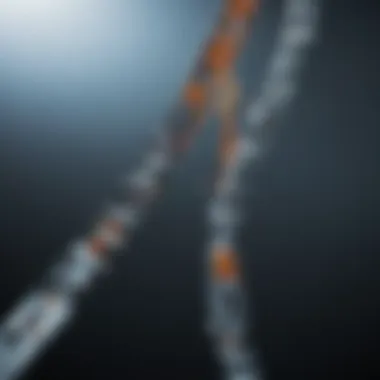
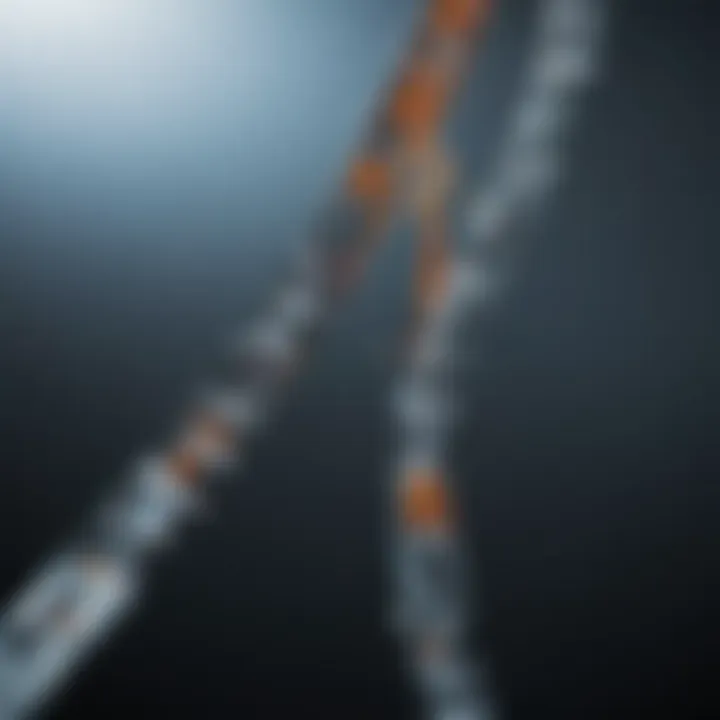
There are two separate strands being replicated: the leading strand and the lagging strand. The leading strand is synthesized continuously, as it runs in the same direction as the replication fork. Conversely, the lagging strand is synthesized in short segments known as Okazaki fragments, which are later connected by DNA ligase.
This stage is not without challenges. Enzymes must work with precision, and any errors may result in mutations, which can lead to various cellular dysfunctions. Thus, the accuracy of the elongation stage is critical for the organism’s wellbeing.
Termination
Termination is the final phase of DNA replication. It occurs when the replication forks meet or when the end of the linear DNA molecule is reached. The completion of the new DNA strands leads to the disassembly of the replication machineries involved.
In eukaryotic cells, specific sequences known as telomeres play a role in the termination process. They protect the ends of chromosomes from degradation. Once the replication is complete, the RNA primers are removed and replaced with DNA. Finally, any remaining gaps are sealed by DNA ligase, ensuring that the two newly formed strands are intact.
In summary, these stages of DNA replication—initiation, elongation, and termination—are crucial for ensuring that genetic information is accurately copied and passed on during cell division. This careful orchestration of molecular machinery is integral to life itself.
Factors Affecting DNA Replication
DNA replication is a complex process, influenced by various factors that can either enhance or hinder its efficiency. Understanding these factors is crucial for comprehending the dynamics of replication and its implications for biology. In this section, we will focus on two primary factors: temperature and pH, as well as nutritional availability. Each of these elements plays a significant role in ensuring that DNA replication occurs accurately and timely.
Temperature and pH
Temperature and pH are critical environmental factors that affect the performance of the enzymes involved in DNA replication. Enzymatic reactions, including those that replicate DNA, are highly sensitive to temperature changes. For instance, the activity of DNA polymerases is optimal at specific temperatures, generally around 37°C in human cells. Deviations from this temperature can lead to reduced activity or even denaturation of the enzyme, causing replication to stall or become erroneous.
The pH level also significantly impacts enzyme functionality. Most enzymes involved in DNA replication, such as DNA polymerase and ligase, operate best within a narrow pH range around 7 to 8. If the pH deviates too far from this optimal range, enzyme activity can decrease. This may lead to improper synthesis of new DNA strands, raising the chance of mutations.
It's essential to maintain cellular homeostasis for these factors. If a cell experiences stressors, such as changes in temperature or pH due to external conditions, its ability to replicate DNA may be compromised. Therefore, organisms have evolved regulatory mechanisms to stabilize these conditions and ensure effective DNA replication.
"Environmental conditions can directly influence biological processes like DNA replication. It is vital to understand their roles for better scientific applications."
Nutritional Availability
Nutritional availability encompasses the essential resources required for cellular processes, including DNA replication. Key nutrients such as nucleotides, vitamins, and minerals directly affect the efficiency and accuracy of the replication process.
Nucleotides, the building blocks of DNA, must be in sufficient supply for replication to proceed. Their absence can lead to incomplete replication cycles, resulting in unstable or fragmented DNA. Furthermore, vitamins like B12 and folate are involved in nucleotide synthesis. A deficiency can directly impair DNA replication by slowing down the availability of these crucial components.
Additionally, minerals such as magnesium play a pivotal role in stabilizing DNA structure during replication. Magnesium ions are co-factors for many enzymes, including DNA polymerases. Without adequate levels of magnesium, enzymes may not function effectively, leading to replication errors.
In summary, the availability of nutrients is vital for proper DNA replication. Cells must have access to these resources to maintain cellular integrity and function. Ensuring an optimal supply of nucleotides and relevant nutrients is integral for life, particularly in rapidly dividing cells.
DNA Repair Mechanisms
DNA repair mechanisms play a crucial role in maintaining the integrity of an organism's genetic material. The continuous exposure to environmental stressors and the cellular metabolism can induce various forms of DNA damage. If left unrepaired, these alterations can result in mutations that lead to diseases, including cancer. Therefore, understanding how these mechanisms work is essential for advancements in molecular biology, medicine, and biotechnology.
Mismatch Repair
Mismatch repair (MMR) is a specific DNA repair process that corrects base-pair mismatches that occur during DNA replication. These mismatches may arise from errors made by DNA polymerase, the enzyme responsible for synthesizing new DNA strands. If not corrected, these mismatches can lead to permanent mutations in the genome.
The MMR process involves several key proteins, including MutS and MutL, which play essential roles in recognizing and repairing errors. When a mismatch is detected, MutS binds to the mismatched DNA site. It then recruits MutL, which helps communicate the error to other proteins that execute the repair. The damaged section of the DNA strand is removed and replaced with the correct nucleotides by DNA polymerase. This mechanism highlights the accuracy of DNA replication and its correction is vital for genetic fidelity.
Mismatch repair ensures that DNA replication fidelity is upheld, significantly reducing the mutation rate in the genome.
Nucleotide Excision Repair
Nucleotide excision repair (NER) is another critical mechanism that repairs bulky DNA lesions and helix-distorting damage. These lesions can result from exposure to ultraviolet radiation or chemical agents, leading to the formation of thymine dimers or other harmful adducts. The NER process is vital for safeguarding genomic stability.
In NER, the damaged DNA is recognized by a complex of proteins that initiate the repair process. First, the DNA is unwound to allow access to the damaged site. A segment of the DNA strand containing the lesion is excised by endonucleases. DNA polymerase then synthesizes new nucleotides to replace the removed segment. Finally, DNA ligase seals the newly formed strand, restoring the integrity of the DNA molecule.
Nucleotide excision repair is essential not only for individual cellular health but also for preventing the accumulation of mutations that may lead to cancer. Its efficiency and effectiveness are indicative of the cell's ability to maintain genomic stability, reflecting the importance of repair pathways in overall health.
Application of DNA Replication in Biotechnology
The applications of DNA replication extend far beyond the basic understanding of biological processes. In biotechnology, the manipulation of DNA replication has empowered various fields such as medicine, agriculture, and forensic science. This section highlights the significance of DNA replication in biotechnology, emphasizing specific methodologies, their advantages, and critical considerations.
Polymerase Chain Reaction (PCR)
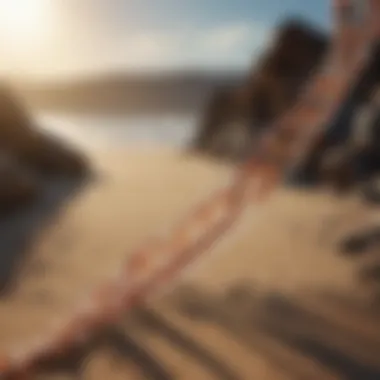
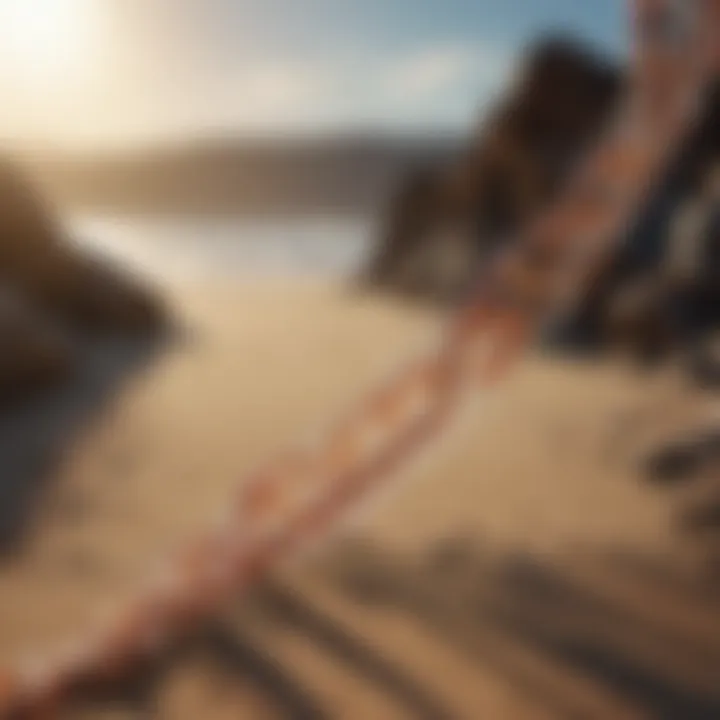
The Polymerase Chain Reaction, commonly known as PCR, is a revolutionary technique that amplifies DNA sequences. PCR relies on the principles of DNA replication, specifically the ability of DNA polymerase to synthesize new strands from a template. PCR allows researchers to create millions of copies of a specific DNA segment in a matter of hours.
The steps involved in PCR include:
- Denaturation: The double-stranded DNA is heated to around 94°C, leading to the separation of the strands.
- Annealing: The temperature is lowered to approximately 54°C, allowing short DNA primers to bind to each strand.
- Extension: DNA polymerase extends the primers, synthesizing new DNA strands, typically at 72°C.
These steps are repeated for 25 to 35 cycles, exponentially increasing the number of copies of the target DNA. PCR is crucial in numerous applications, including:
- Medical Diagnostics: Detecting pathogens in diseases by amplifying their genetic material.
- Genetic Research: Studying genes, mutations, and evolutionary biology by providing ample genetic material for analysis.
- Forensic Science: Analyzing DNA evidence from crime scenes for identification purposes.
This technique has revolutionized molecular biology, enabling scientists to conduct research and diagnostics with great efficacy and precision.
Genetic Engineering
Genetic engineering encompasses a wide array of techniques that modify the genetic structure of organisms. DNA replication plays a vital role in genetic engineering, which often involves the manipulation of DNA sequences for improved traits or functionalities. For example, inserting a gene of interest into a plasmid relies on understanding how DNA replicates.
Common applications of genetic engineering include:
- Agricultural Biotechnology: Developing genetically modified organisms that have enhanced resistance to pests, diseases, or environmental conditions. This can lead to higher crop yields and nutritious food production.
- Gene Therapy: Inserting therapeutic genes into human cells to correct genetic disorders. This requires precise replication of both the therapeutic gene and related regulatory sequences.
- Synthetic Biology: Designing and constructing new biological parts or systems through engineered DNA replication. This can lead to innovative solutions in biofuel production or sustainable materials.
While the benefits of genetic engineering are substantial, ethical considerations remain critical. Issues relating to biosafety, environmental impact, and bioethics should be continuously assessed to ensure responsible and beneficial use of technology.
Through understanding DNA replication, biotechnologists can advance research and practical applications that have far-reaching effects on society.
Recent Advances in DNA Replication Research
Recent advances in the field of DNA replication research have brought a wealth of knowledge that is crucial for both fundamental biology and applied sciences. These developments not only enhance our understanding of cellular processes but also open new avenues for innovation in genetic engineering and therapeutics. Studying the meticulous nature of DNA replication helps us uncover the mechanisms behind various diseases, genetic disorders, and potential solutions for these problems.
One notable focus of this research has been on the role of novel enzymes and the intricate protein interactions that facilitate replication. Insights gained from studying these factors enable scientists to tailor genetic modifications with higher precision. The implications span multiple sectors, including medicine, agriculture, and synthetic biology.
"Each discovery leads to greater comprehension of life at its most fundamental level, informing us about cellular functions and guiding practical applications."
CRISPR Technology
CRISPR technology represents a revolutionary step in genetic engineering, stemming from an understanding of DNA replication and repair mechanisms. CRISPR-Cas9 allows for targeted modifications in the genome, and this approach relies on the precise functionality of various replication enzymes. Scientists harness this technology to edit genes, remove defects, or incorporate beneficial traits into organisms.
The efficiency of CRISPR can enhance gene therapy's effectiveness and minimize off-target effects. This becomes particularly important in treating genetic diseases, where traditional methods may encounter limitations. Researchers continue to explore and refine CRISPR techniques, leading towards more efficient and safer applications in human health treatments and beyond.
Synthetic Biology Applications
Synthetic biology is another intriguing outcome of advancements in DNA replication research. This interdisciplinary field combines biology and engineering principles to design and construct new biological parts, devices, and systems. Understanding the fundamentals of DNA replication enhances the designs of synthetic organisms to perform specific tasks.
Some current applications include:
- Creating improved biofuels
- Developing biosensors for pollution detection
- Engineering microorganisms for pharmaceutical production
- Constructing novel pathways for metabolic engineering
Research in DNA replication helps refine tools for creating synthetic organisms by understanding how molecular mechanisms can replicate and recombine existing biological systems. This understanding might lead to more sustainable processes and innovative approaches to solving global challenges, such as food production and energy generation.
Ending
In any comprehensive analysis or exploration, the conclusion is a vital component. Here, it consolidates the knowledge acquired, emphasizing the core themes discussed throughout the article. This important section delves into the significance of DNA replication, highlighting its necessity for cellular function, genetic continuity, and the implications of recent advances in molecular biology.
DNA replication is not merely a biological event; it ensures the propagation of genetic material, thus sustaining life across generations. Understanding this process equips students, researchers, and professionals with insights into fundamental biological mechanisms.
Summary of Key Points
- DNA replication is an essential process, enabling the accurate duplication of genetic material.
- The semi-conservative nature of replication ensures that each daughter strand contains one original and one newly synthesized strand.
- Key enzymes like DNA helicase, polymerase, ligase, and primase play crucial roles in the replication process.
- Factors such as temperature, pH, and nutrient availability can impact replication efficiency and accuracy.
- Advances in biotechnology, such as PCR and CRISPR, demonstrate the practical applications of DNA replication knowledge.
Future Directions in Research
The field of DNA replication research is continually evolving. Future directions might focus on the following:
- Understanding Error Correction: Deeper insights into the mechanisms of proofreading and repair might yield breakthroughs in genetics and medicine.
- Therapeutic Applications: Exploring how replication processes can be harnessed in gene therapy to address hereditary conditions or cancer.
- Synthetic Biology: Investigating synthetic mechanisms that can enhance or redesign DNA replication for novel applications in biotechnology.
- Environmental Impacts: Studying how external changes, such as climate variations, affect the replication processes in various organisms.
The continuous unfolding of DNA replication research holds the potential to revolutionize multiple scientific fields. It can help us gain further knowledge and solve complex biological problems.
"The elegance of DNA replication illustrates the delicate balance of life, showcasing both precision and complexity."