Scintillator Radiation Detectors: Mechanisms and Uses
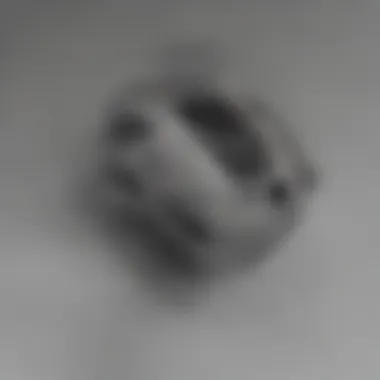
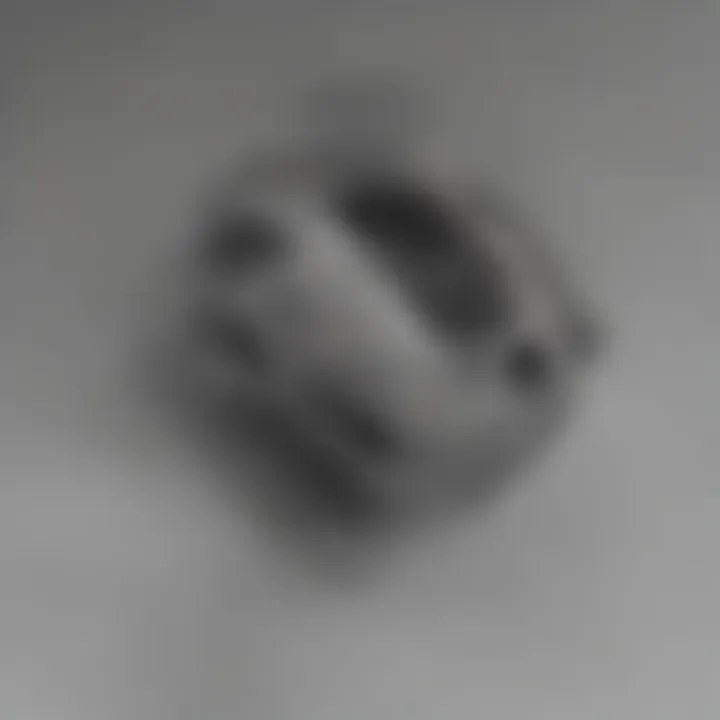
Intro
Scintillator radiation detectors represent a vital technology in various fields, including medical imaging, nuclear physics, and environmental monitoring. They convert high-energy radiation into visible light, which can then be detected and quantified. Understanding the core principles and advancements of scintillator detectors is essential for students, researchers, educators, and professionals who work in radiation detection and related applications. This section will explore the underlying technologies of scintillators along with the significant advancements pushing this field forward.
Research Methodology
Description of research design and approach
The examination of scintillator radiation detectors involves both theoretical underpinnings and empirical assessments. A multi-faceted approach is often employed, blending experimental measurements with computational simulations. This hybrid methodology allows for a detailed exploration of how different scintillator materials respond to varying radiation sources. Researchers deploy a range of statistical analysis methods to analyze the efficiency and performance of these detectors against existing benchmarks.
Materials and methods used in the study
Researchers typically utilize various types of scintillator materials, such as organic crystals, inorganic crystals like sodium iodide, and plastic scintillators. These materials are chosen based on specific applications, performance characteristics, and cost-effectiveness. In laboratory settings, the experimental setup commonly includes:
- Radiation sources for testing: isotopes or X-ray machines.
- Photodetectors: devices like photomultiplier tubes (PMTs) or silicon photomultipliers (SiPMs) that convert the light emitted by scintillators into an electrical signal.
- Data acquisition systems for capturing the signal outputs for further analysis.
- Shielding materials to isolate experiments from background radiation noise.
This structured approach ensures that researchers can draw valid conclusions about the efficacy and operational capabilities of various scintillator detectors.
Discussion and Interpretation
Interpretation of results in the context of existing literature
Findings from recent studies indicate diverse performance levels based on scintillator composition and detector design. Notably, sodium iodide's efficiency remains unparalleled for gamma-ray detection, while plastic scintillators offer advantages in terms of cost and versatility. Research suggests that hybrid designs, integrating different scintillator materials, can enhance overall detector capabilities. These advancements align well with the ongoing trend of improving detection systems to meet the demand of various industries.
Implications for future research or practical applications
As technology progresses, the importance of scintillator radiation detectors is set to expand. There is a growing need for more efficient, compact, and cost-effective solutions in sectors ranging from health care to nuclear security. Future research should focus on developing new scintillator materials and improving existing designs to enhance sensitivity and reduce detection limits. Integration with digital technologies is also a crucial area that could yield novel applications in real-time radiation monitoring.
Scintillator detectors are not just tools; they are gateways to understanding and exploring the behavior of radiation, making them irreplaceable in modern science.
Preface to Scintillator Radiation Detectors
Scintillator radiation detectors play a crucial role in various fields, including medical imaging, nuclear safety, and scientific research. Their ability to efficiently detect and measure radiation makes them valuable tools in modern technology. This section provides insight into the significance and functionality of scintillator detectors, establishing a foundation for understanding their principles and applications.
Definition and Purpose
A scintillator is a substance that exhibits luminescence when it absorbs ionizing radiation. When radiation interacts with the scintillator material, it creates excited states in the atoms of the material. These excited states subsequently release energy in the form of visible light. The primary purpose of scintillator detection is to facilitate the measurement of radiation levels, providing critical data for various applications. Scintillator detectors are prized for their high sensitivity, fast response time, and the ability to differentiate between different types of radiation. This capacity to convert high-energy radiation into detectable light is essential for accurate readings in many industries, ranging from healthcare to security.
Historical Background
The evolution of scintillator detectors dates back to the early 20th century, closely tied to advancements in nuclear physics. The first documented scintillator, the sodium iodide crystal, became widely used in the 1940s for its excellent scintillation properties. Researchers recognized its utility for detecting gamma radiation, paving the way for further innovations in detector technology. Over the decades, scintillator materials have diversified, incorporating organic and inorganic compounds. Each material exhibits distinctive properties that influence its application and efficiency. The development of photomultiplier tubes further revolutionized scintillation detection by significantly enhancing light collection and conversion capabilities.
This history illustrates not only the growth in detection technologies but also their importance in a wide range of scientific fields. Understanding the past leads to appreciation of current advancements and their potential future impact. Scholars and engineers continue to explore innovative materials and designs to improve the functionality and versatility of scintillator radiation detectors.
Fundamental Principles of Scintillation
The fundamental principles of scintillation are critical for understanding how scintillator detectors function. The efficiency and accuracy in radiation detection rely on these underlying mechanisms and processes. Insight into scintillation facilitates improvements in detector design, making them applicable in diverse fields like medical imaging and scientific research. The principles dictate how materials respond to ionizing radiation, converting it into measurable light.
Mechanisms of Scintillation
Scintillation occurs when a scintillator material absorbs high-energy radiation and re-emits it as visible light. This process begins with the incident radiation striking the material, causing ionization. As the material reacts, it generates excited states. These states eventually return to a lower energy state, releasing energy as photons in the process.
There are two primary mechanisms at work here:
- Direct Interaction: In some scintillators, such as inorganic crystals like Sodium Iodide doped with Thallium, the radiation directly excites electrons within the lattice structure. This creates excitons, which then dissociate to emit light.
- Energy Transfer: Certain materials, particularly organic scintillators, rely on the transfer of energy from a primary excited state to secondary species, facilitating light emission.
This duality illustrates the complexity and variability in scintillator mechanisms. Materials used in scintillators must be carefully chosen for these interactions to be efficient, as their properties directly affect detector performance.
Energy Transfer Processes
Energy transfer processes play a vital role in the scintillation mechanism. They describe how energy from ionizing events is transported within the scintillator material before photon emission occurs. Typically, this involves two key types of interactions:
- Radiative Energy Transfer: This process involves the transfer of energy through radiation of electromagnetic waves, which can lead to the generation of photons.
- Non-Radiative Energy Transfer: In this case, energy is transferred directly from one excited molecule to another without the emission of photons. This is often efficient in organic scintillators where molecular interactions are prevalent.
Understanding these energy transfer processes is essential for enhancing the design of solid materials and developing new scintillator types. Improvements in these areas can lead to advancements in energy resolution and timing performance for detector systems, making scintillator detectors more effective in their applications.
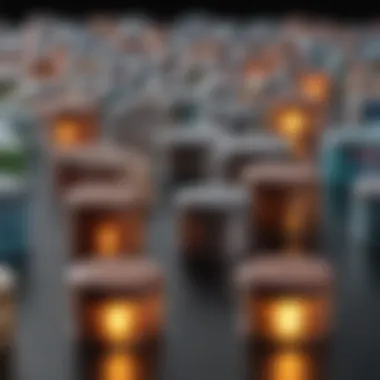
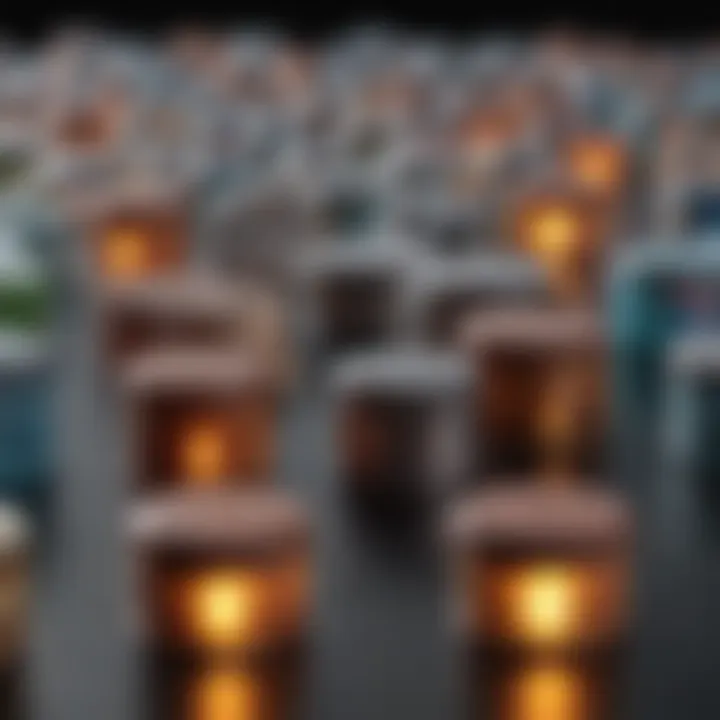
A strong understanding of the mechanisms and energy transfer in scintillation allows researchers to tailor scintillator materials for specific applications, optimizing their performance for precise measurements.
Types of Scintillators
Understanding the different types of scintillators is crucial in the study and application of scintillator radiation detectors. Each type has unique properties and benefits that can influence performance in various settings. Selection criteria often involve the energy range of interest, the required resolution, and environmental considerations. The efficiency and effectiveness of a scintillator detector depend heavily on the specific type of scintillator material used.
Organic Scintillators
Organic scintillators are made from carbon-based materials. They are often considered for their high light output and fast response time, which makes them suitable for detecting fast-moving particles and radiation. Typically, these scintillators include aromatic compounds and plastics with embedded additives to enhance their performance. Their flexibility in design and the ability to produce large-area detectors are significant advantages.
Benefits of Organic Scintillators:
- High Resolution: They generally provide good energy resolution, allowing for precise measurements of incoming radiation energies.
- Fast Timing: The response time is quick, allowing for effective timing measurements of radiation events.
- Cost-Effectiveness: Organic scintillators tend to be less expensive to produce compared to inorganic types.
A common example of an organic scintillator is the use of stilbene, which has been widely used for neutron detection in various applications. The choice of material can impact the overall detection system's performance, and ongoing research continues to refine the efficiency of organic scintillators.
Inorganic Scintillators
Inorganic scintillators, usually composed of crystals, are known for their robustness and superior light yield compared to their organic counterparts. These materials often include compounds such as sodium iodide doped with thallium or cesium iodide. Inorganic scintillators are widely used in medical imaging and nuclear applications due to their excellent energy resolution and durability.
Benefits of Inorganic Scintillators:
- High Light Output: These materials typically have a higher light output than organic scintillators, making them highly efficient for gamma-ray detection.
- Durability: Their solid structure ensures better resilience against radiation damage and environmental conditions.
- Superior Energy Resolution: Inorganic scintillators often provide improved energy resolution, especially with applications where precise energy measurement is critical.
For instance, sodium iodide detectors are commonly used in gamma spectroscopy due to their excellent performance. The efficiency and clarity of the signals generated by inorganic scintillators facilitate accurate analysis essential in fields like nuclear medicine and security.
Plastic Scintillators
Plastic scintillators represent a versatile category that combines the advantages of organic and inorganic materials. They are primarily made from polymer matrices that can incorporate scintillating compounds. This type has gained attention due to its lightweight, flexibility, and ability to be fabricated in diverse shapes and sizes.
Benefits of Plastic Scintillators:
- Lightweight and Flexible: The material ease of handling and installation makes plastic scintillators suitable for a variety of settings and configurations.
- Customizable Forms: Their manufacturing process allows for the design of unique shapes needed for specific applications.
- Cost Efficiency: Generally more economical than crystal scintillators, making them accessible for diverse projects.
Plastic scintillators are often utilized in radiation detection systems that require portable setups, such as field detectors and personal dosimeters. Their adaptability and affordability continue to make them a popular choice among researchers and practitioners alike.
In sum, the type of scintillator selected directly influences the performance metrics of radiation detectors. Selecting the right material based on application needs is paramount for achieving desired detection outcomes.
Detector Design and Engineering
The design and engineering of scintillator detectors is a fundamental aspect that greatly influences their performance and applicability. The efficiency, reliability, and overall functionality of these detectors stem from careful consideration of various design elements. This section outlines key components of detector design, emphasizing their significance in ensuring optimal performance.
Geometric Considerations
Geometric considerations play a crucial role in the development of scintillator detectors. The shape and dimensions of a detector directly impact its ability to capture radiation efficiently.
- Volume and Shape: The volume of the scintillator material affects the probability of interactions with incoming radiation. For instance, larger volumes may increase detection efficiency, yet they can also introduce complications such as light loss due to scattering.
- Surface Area: A larger surface area can enhance the likelihood of photon emission, which is essential for effective light collection by the photodetector.
- Geometry and Shielding: The geometric configuration must consider the placement of shielding materials to minimize background noise and improve signal clarity. A well-designed shield ensures that only relevant signals from radiation sources are detected, enhancing the signal-to-noise ratio.
These geometric elements are crucial for optimizing the detector's overall response and can significantly influence the design decisions made during the engineering process.
Photodetector Coupling
Photodetector coupling is another essential aspect of scintillator detector design. This refers to the interface between the scintillator material and the photodetector, which converts the light generated by scintillation into an electrical signal.
- Types of Photodetectors: Common types of photodetectors used include photomultiplier tubes (PMTs) and silicon photomultipliers (SiPMs). Each type has its advantages and disadvantages regarding sensitivity, size, and operational conditions.
- Coupling Methods: The coupling method employed influences the efficiency of light collection. Optical coupling methods such as using optical gels or adhesives can enhance light transmission between the scintillator and the photodetector.
- Alignment and Geometry: Proper alignment between the scintillator and the photodetector is critical. Any misalignment can lead to a significant loss of detected signals, ultimately degrading the detector's performance.
Effective coupling between the scintillator and the photodetector plays a key role in optimizing detection capabilities. A robust engineering approach is necessary for ensuring maximum signal output.
Performance Metrics for Scintillator Detectors
The performance metrics for scintillator detectors are crucial for assessing how effectively these devices function. Understanding these metrics helps in determining the suitability of a detector for specific applications. Key performance metrics include energy resolution and timing resolution. These aspects are essential because they directly influence the ability to detect, identify, and measure radiation with precision.
Energy Resolution
Energy resolution refers to a scintillator detector's ability to distinguish between different energy levels of incoming radiation. Essentially, it measures how well a detector can resolve the energy of an incident gamma-ray or similar particles. High energy resolution is important in applications like spectroscopy, where precise energy measurements are needed to identify isotopes.
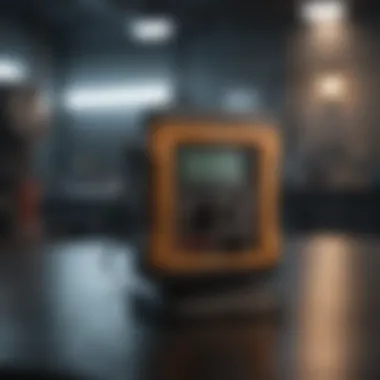
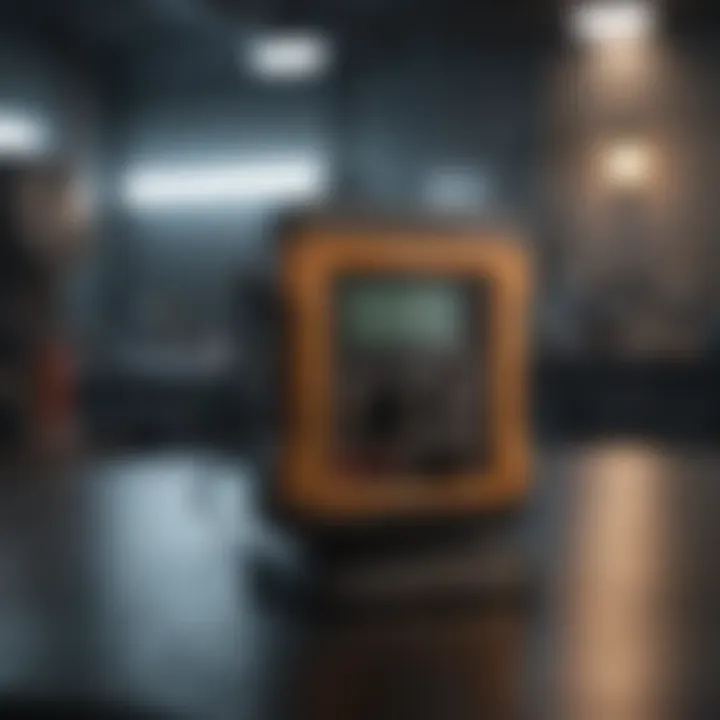
An energy resolution of less than 3% is typically desirable for most applications. This capability allows researchers to differentiate between closely spaced energy peaks in a spectrum. Factors affecting energy resolution include the material properties of the scintillator, the quality of the photodetector, and the signal processing methods employed.
- Material Factors: The purity and composition of the scintillator material can significantly impact energy resolution. For instance, cesium iodide (CsI) provides better energy resolution compared to sodium iodide (NaI), albeit at a higher cost.
- Photodetector Quality: Devices like photomultiplier tubes or silicon photomultipliers can introduce noise and affect the measured energy. The choice of detector plays a pivotal role in achieving optimal energy resolution.
- Signal Processing: Advanced algorithms for signal processing can further enhance energy measurement accuracy. Use of digital filtering can help minimize noise and improve the clarity of detected signals.
Timing Resolution
Timing resolution is another vital metric, defining how accurately a scintillator detector can timestamp an event. High timing resolution is critical in applications where timing is essential, such as particle physics experiments or time-of-flight measurements. A good timing resolution allows for the effective discrimination of pulses, which is particularly crucial in environments with many concurrent events.
Typically, timing resolutions in the range of a few nanoseconds are required for high-energy physics applications. The importance of timing resolution can be outlined by its essential parameters:
- Event Localization: A precise timing resolution helps in localizing the position of a radiation event in space, aiding in the construction of complex particle tracks.
- Pulse Shape Analysis: Different interactions in a scintillator can produce different pulse shapes. An effective timing resolution permits detailed analysis of these pulse shapes, giving insight into the type of interaction that took place.
- System Optimization: Improving timing resolution involves optimizing both the detector design and readout electronics. Implementing faster electronics and better signal amplification strategies directly enhances timing precision.
In summary, the performance metrics of energy and timing resolution are foundational to the effective use of scintillator detectors. They inform the design choices and operational parameters of detectors aiming for improved sensitivity and accuracy across a wide range of applications.
"The capacity to measure energy and time with remarkable precision allows scintillator detectors to play an invaluable role in scientific research and practical applications."
These performance aspects are not just technical specifications; they guide the application of scintillator detectors in fields like medical imaging and nuclear safety. Enhancing these metrics remains a crucial focus for ongoing research and development.
Applications of Scintillator Detectors
The applications of scintillator detectors span a wide range of fields, highlighting their versatility and effectiveness. These detectors have become a cornerstone in different industries, where precise measurements are crucial. Their capability to capture and convert ionizing radiation into visible light offers numerous advantages in applications such as medical imaging, nuclear power, homeland security, and scientific research. In this section, we will explore specific applications, examining the benefits each brings to its respective field.
Medical Imaging
In the medical sector, scintillator detectors play a vital role in imaging technologies like positron emission tomography (PET) and single-photon emission computed tomography (SPECT). These imaging techniques rely on the ability of scintillators to detect gamma rays emitted from radioactive tracers injected into patients.
Key Aspects of Medical Imaging Use
- Higher Sensitivity: Scintillator detectors show enhanced sensitivity to gamma rays, allowing for more accurate imaging and diagnosis.
- Real-time imaging capability: The rapid response of these detectors contributes to dynamic imaging, essential in monitoring physiological processes.
- Dose Reduction: Improved detection efficiency leads to a potential reduction in radiation dose received by patients, enhancing safety.
Applications in medical imaging not only enable effective diagnostics but also improve treatment planning for various conditions, such as cancer.
Nuclear Industry
In the nuclear industry, scintillator detectors are fundamental for operational safety and regulatory compliance. They are used to monitor radiation levels in nuclear power plants and during the handling of radioactive materials.
Important Functions in Nuclear Industry Include
- Radiation Monitoring: Continuous monitoring helps manage the safety of workers and the environment.
- Material Identification: Scintillator detectors can identify specific isotopes, aiding in waste management and material control.
- Accident Response: In the event of a radiation leak, these detectors are crucial for rapidly assessing exposure levels, guiding response actions.
Their use in this sector underscores both operational efficiency and environmental safety.
Homeland Security
In the context of homeland security, scintillator detectors are critical tools for detecting illicit radioactive materials and safeguarding sensitive locations. Their ability to detect gamma rays has led to their deployment in various security applications.
Considerations in Homeland Security Applications
- Port Security: Deployments in ports help scan shipping containers for unauthorized nuclear materials.
- Border Security: Mobile detectors can assess vehicles and individuals crossing borders, enhancing national security.
- Event Security: Public events can utilize scintillator detectors to detect radiological threats, ensuring safety for large gatherings.
Their importance in ensuring public safety and national security cannot be overstated.
Scientific Research
The field of scientific research also benefits significantly from scintillator detectors, particularly in high-energy physics and astrophysics. These detectors are used in experiments to understand fundamental particles and cosmic phenomena.
Applications in Research
- Particle Detection: Scintillators are integral in accelerators, where they help detect high-energy particles produced in collisions.
- Astrophysical Measurements: They can measure cosmic radiation, aiding in research about the universe.
- Nuclear Physics: In studies involving nuclear decay and reactions, scintillator detectors provide essential data.
Their utility within research underscores their role in advancing scientific knowledge.
Scintillator detectors are more than just instruments; they are crucial to advancements across multiple fields, enhancing our understanding and safety in medical, industrial, and research settings.
Recent Advancements in Scintillator Technology
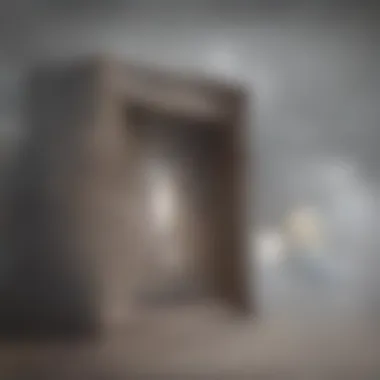
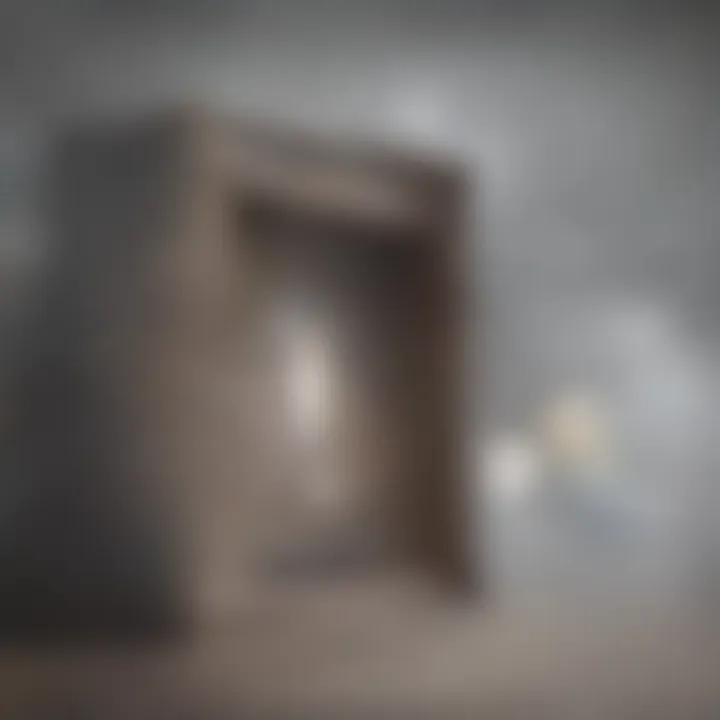
Scintillator technology is crucial for improving detection efficiency in various fields such as medical imaging and safety. These advancements not only enhance detection capabilities but also drive innovation in materials and system designs. With ongoing research and development, scintillator detectors are becoming more versatile and effective.
Hybrid Detector Systems
Hybrid detector systems represent a significant leap forward in the use of scintillator technology. By combining different detection methods, these systems can achieve higher efficiency and precision. For instance, integrating scintillators with semiconductor detectors allows for better energy resolution. This combination reduces noise and increases the quality of detected signals.
The advantages include:
- Improved energy resolution
- Faster response times
- Greater detection range
- Enhanced capability to differentiate between types of radiation
Hybrid systems are particularly useful in medical and industrial applications, where the ability to accurately distinguish between various radiation types is essential. This dual approach not only enhances performance but also expands the operational range of scintillator detectors.
Novel Materials Research
Research into novel materials is transforming scintillator technology. New materials can lead to significant enhancements in sensitivity and response times. Recent studies have focused on inorganic compounds, such as cesium iodide, and advanced organic materials.
The benefits of using these novel materials include:
- Higher light output
- Improved energy resolution
- Enhanced radiation hardness
- Better scalability for different applications
Researchers are exploring the use of nanostructures and composite materials, which may offer better performance metrics and lower costs. Ongoing investigations in this area show a promise for notable breakthroughs in future scintillator applications.
"The future of scintillator technology is closely tied to advancements in materials science, opening new avenues for practical applications in detection technology."
Challenges in Scintillator Detection
Scintillator detectors play a crucial role in various fields such as medical imaging and radiation detection. However, these devices face several challenges that can compromise their effectiveness. Understanding these challenges is vital for advancing technology and improving performance.
Radiation Damage
Radiation damage is a significant challenge for scintillator detectors. The interaction of high-energy radiation with scintillator materials can lead to a variety of issues. Over time, the crystal structure may change, leading to reduced light output and efficiency. This degradation can affect the detector's ability to precisely measure radiation levels. Moreover, the materials used in scintillators, especially organic ones, tend to be more susceptible to radiation damage. This introduces a need for materials that can withstand intense radiation while maintaining their performance. Research into radiation-resistant materials is ongoing and is essential for the future reliability of scintillator detectors.
Signal Interference
Signal interference poses another challenge in scintillator detection. Background radiation and electronic noise can distort signals, making it difficult to accurately interpret data. This interference can arise from various sources, including nearby electronic devices or environmental radiation. Additionally, the way the scintillator is coupled to photodetectors can also influence the signal quality. Proper shielding and noise reduction techniques are critical in mitigating these interferences. Advanced signal processing techniques are being developed to improve diagnosis accuracy and enhance signal clarity. Ensuring high-quality signals is paramount for effective radiation detection.
Effective management of these challenges is key to optimizing scintillator technology. By addressing radiation damage and signal interference, researchers can enhance detector performance and reliability.
Future Directions in Scintillator Research
The field of scintillator radiation detectors is ever-evolving, with research opportunities expanding in various directions. Understanding the future directions in scintillator research is crucial for harnessing advanced technologies that can enhance the capabilities of these detectors. As we look ahead, several elements stand out, including emerging applications in multiple industries and the development of innovative designs that promise improved performance. These advancements not only improve detection efficiency but also help meet the demands of modern scientific research and practical applications.
Emerging Applications
Scintillator detectors are finding new uses across a range of sectors, extending well beyond traditional nuclear industry roles. Medical imaging is one notable area where scintillators are taking center stage. For instance, the integration of advanced scintillator materials in PET scanners enhances image quality and reduces radiation doses for patients. Another promising application is in environmental monitoring, where these detectors can identify low levels of radiation in various settings, contributing to safety and regulatory compliance.
In the military and defense sector, scintillator detectors are essential for detecting clandestine activities involving nuclear materials. Moreover, the field of physics research is continually benefiting from scintillator advancements, especially in high-energy experiments such as those conducted at CERN. The ability to accurately measure radiation in particle physics experiments exposes new frontiers in understanding fundamental particles and forces.
New applications also involve industrial radiography, with scintillator detectors being employed for non-destructive testing. Here, they ensure product integrity and safety in highly regulated industries like aerospace. These emerging applications demonstrate that scintillator technology is not static; it is dynamic and responsive to contemporary challenges.
Innovative Designs
As the demand for more efficient and sensitive detectors rises, innovative designs are becoming a focal point of research. These designs prioritize enhancements in material performance, where new compounds for scintillation materials are being explored. For example, the development of nanocrystalline scintillators has shown promise in achieving higher light output and faster response times, making them ideal for applications requiring real-time detection.
Moreover, advancements in photon detection technologies are significantly impacting scintillator designs. The integration of advanced photodetectors, such as silicon photomultipliers (SiPMs), into scintillator systems improves light collection efficiency and energy resolution. These innovations lead to more compact and robust detector systems that can be deployed in harsher environments, increasing their practical utility.
Research is also being directed toward multi-modal detector systems that combine scintillation with other detection principles, such as semiconductor detection technologies. This hybrid approach can leverage the best aspects of each modality, offering improved accuracy and flexibility.
Closure
The conclusion of this article serves as a pivotal point that encapsulates the major insights gained through the exploration of scintillator radiation detectors. It reinforces the critical role these detectors play in various fields, from medical imaging to nuclear security. Moreover, it examines key factors that highlight the significance of scintillators in advancing both scientific knowledge and practical applications.
Summary of Key Points
In reviewing the text, several crucial aspects stand out:
- Definition and Purpose: Scintillator detectors are unique tools that convert high-energy radiation into visible light, facilitating the detection and measurement of radiation thresholds.
- Variety of Types: This article discusses the diversity of scintillator materials—organic, inorganic, and plastic—and their respective advantages in terms of efficiency and appropriate application contexts.
- Technological Advances: Hybrid detector systems and new material research have been identified as critical areas influencing the performance metrics of scintillator detectors.
- Applications: Various applications ranging from medical diagnostics to homeland security highlight the extensive utility of scintillator detectors in real-world scenarios.
Implications for the Future
The advancements in scintillator technology suggest a continuously evolving landscape. Emerging applications could lead to breakthroughs in detecting weaker signals while minimizing interference from background sources. Novel designs could address current challenges, such as radiation damage and component degradation over time. Furthermore, the integration of artificial intelligence in signal processing holds potential for enhancing detection capabilities in the future.