Understanding Enzymes: Their Composition and Role
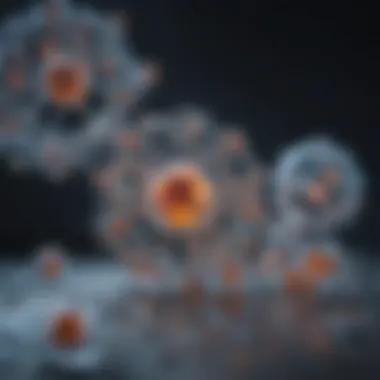
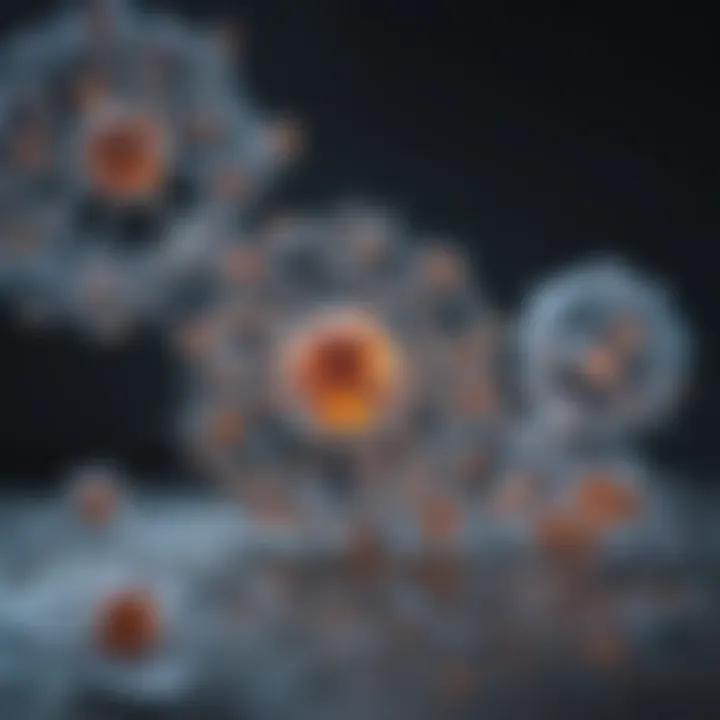
Intro
Enzymes are more than just buzzwords in biology; they are the unsung heroes behind numerous biochemical reactions that keep life ticking. These remarkable proteins act as catalysts, speeding up processes that would otherwise occur at an imperceptibly slow pace. From digestion to DNA replication, enzymes play essential roles in maintaining the delicate balance of life. This piece delves into the composition of enzymes, the molecular structures that give rise to their functionality, and the broader implications for biotechnology and medicine, illuminating how these biomolecules are fundamental to both basic science and applied research.
Research Methodology
When investigating the complex world of enzymes, a structured research methodology is fundamental.
Description of Research Design and Approach
The research approach embraced a multifaceted design, focusing on both theoretical frameworks and practical applications. A combination of quantitative analysis and qualitative insights was employed to reach a comprehensive understanding. By evaluating existing literature, experimental studies, and various case reports, a solid foundation was established. This synthesis revealed how various enzyme compositions directly influence their effectiveness as catalysts.
Materials and Methods Used in the Study
Multiple sources provided the basis for the research, including:
- Peer-reviewed journals: Articles and studies detailing enzyme mechanisms, including publications from renowned journals such as Nature and Biochemistry.
- Textbooks: Foundational literature that outlines enzyme classification, structure, and function.
- Databases: Online resources like en.wikipedia.org and britannica.com were referenced for comparative analysis and real-world examples, validating theoretical concepts with practical instances.
The research also utilized software for modeling enzyme structures, enabling visual interpretations of their active sites and binding affinities.
Discussion and Interpretation
The results gleaned from the research highlighted several pivotal themes in enzyme functionality. It became clear that the intricate structures of enzymes are precisely tailored for their specific functions. For instance, the active sites of enzymes are shaped in such a way that only specific substrates fit, much like a key in a lock.
Important Insight: This specificity is paramount; it ensures that enzymes catalyze the correct chemical reactions while minimizing side reactions. This aligns with the principles observed in previous studies and underscores how even slight alterations in amino acid sequences can significantly affect enzyme efficiency.
Interpretation of Results in the Context of Existing Literature
Through careful analysis, it is evident that existing literature supports the findings of this study. Many researchers have documented how factors such as temperature, pH, and the presence of cofactors can alter enzyme functionality, corroborating earlier theories about enzymatic catalysis and biochemistry.
Implications for Future Research or Practical Applications
Understanding enzyme composition paves the way for innovative advancements in biotechnology and pharmaceutical development. For example, enzymes engineered for specific tasks can improve efficiency in industrial processes, and the design of enzyme inhibitors can lead to new drug therapies. Future research could explore the genetic engineering of enzymes for tailored applications, opening doors to enhanced therapeutic strategies against various diseases.
Foreword to Enzymes
Enzymes are pivotal players in the realm of biochemistry, serving as the heroes that facilitate essential reactions in living organisms. Without them, life as we know it wouldn't exist, as biochemical processes would grind to a halt. This article seeks to demystify the complex world of enzymes, unpacking their intricate composition and the multitude of functions they perform within biological systems.
The Importance of Enzymes
To grasp the significance of enzymes, one must first understand their role as catalysts. Simply put, catalysts are substances that accelerate chemical reactions without undergoing any permanent changes themselves. In the context of enzymes, their protein-based design allows them to lower the activation energy necessary for reactions, thereby speeding up processes that would otherwise be far too slow to sustain life. The majority of metabolic pathways in organisms rely heavily on enzymes, underscoring their importance in not just internal reactions but also in processes like digestion, energy production, and cellular signaling.
Key Elements in Enzyme Functionality
The functionality of enzymes is directly tied to their unique composition. Enzymes are primarily made up of amino acids, the fundamental building blocks of proteins. The way these amino acids are sequenced and folded into specific structures determines how enzymes interact with their substrates, the molecules they act upon. This specificity is also influenced by additional components that might assist enzymes, known as cofactors and coenzymes. Understanding these elements is crucial for both scientific research and applied fields, like drug development, where specific enzyme inhibitors can be targeted for therapeutic benefits.
"Enzymes are nature's best friends, shaping the footsteps of life through every reaction they catalyze."
Benefits of Understanding Enzymes
A deep dive into enzymes not only educates one on their importance in natural processes but also opens doors to numerous applications in biotechnology and pharmaceuticals. By grasping how enzymes work and what influences their activity, researchers can develop novel solutions for various challenges, from environmental cleanup to medical advancements.
In sequencing this article, readers will be guided through the various layers of enzyme composition—right from their definitions to historical insights. This roadmap will facilitate a comprehensive understanding of how enzymes have evolved, their multi-faceted roles in contemporary science, and their potential future applications. In this way, the journey of understanding begins with the basics, providing the reader with a solid foundation to appreciate the dynamic world of enzymes.
The Molecular Constitution of Enzymes
Understanding the molecular constitution of enzymes is crucial to grasping the breadth of their functionalities and applications. Enzymes are complex proteins that facilitate countless biochemical reactions within living organisms by lowering activation energy. They possess structures that define their roles, starting from their primary sequence all the way up to quaternary formations.
The way enzymes are constructed not only determines their efficiency as catalysts but also highlights how the slightest alterations to their architecture can significantly impact their activity. This section delves into the various structural layers of enzymes, emphasizing the significance of each level and how they interconnect to contribute to enzyme functionality.
Primary Structure: Amino Acids
Peptide Bonds
Peptide bonds form the backbone of all proteins, including enzymes. This bond links the amino group of one amino acid to the carboxyl group of another, resulting in a chain. The strength of peptide bonds is a key aspect of enzyme stability and function, making them a popular choice in biological systems.
Each peptide bond exhibits a rigid nature, limiting rotation and effectively flattening peptide chains into a stable structure. This rigidity reinforces the unique sequential order of amino acids, which encode the specific characteristics of different enzymes.
However, there is a downside; the inflexibility of peptide bonds could limit the adaptability of certain enzymes under varying conditions. Nevertheless, their fundamental role in forming the primary structure is irreplaceable in a wide array of biochemical functions.
Amino Acid Variability
Amino acid variability directly affects the enzyme's overall functionality. The differing side chains, or R groups, that amino acids display allow for a vast array of interactions and configurations, leading to a diverse range of enzyme properties. This variability is a beneficial feature, as it grants enzymes their specificity and unique capabilities in different biological contexts.
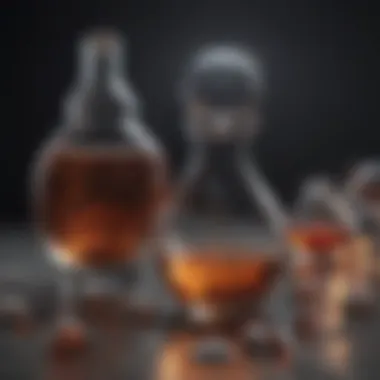
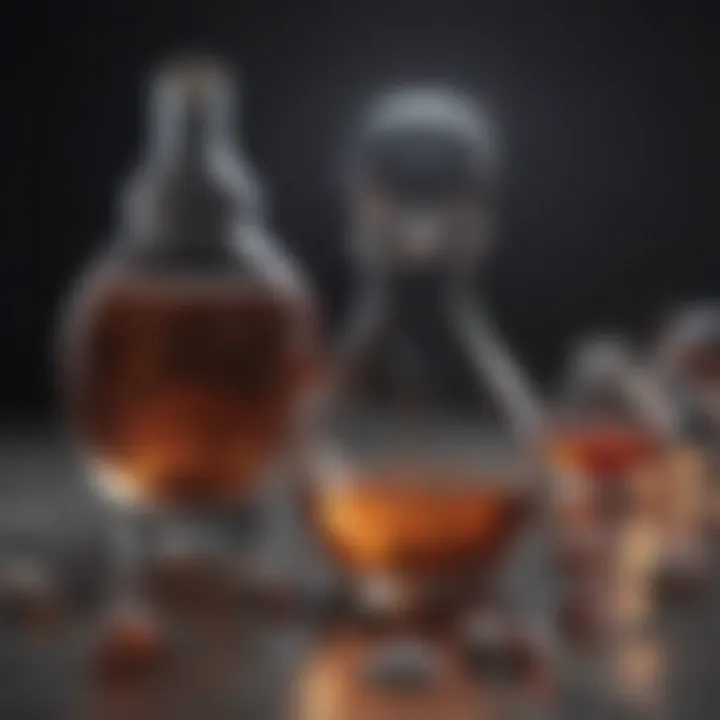
The ability to swap one amino acid for another can lead to new functions or a total loss of activity. Thus, this variability is both advantageous and disadvantageous; while it enriches enzyme function diversity, it can also mean that slight changes can drastically affect reaction pathways.
Secondary Structure: Folding Patterns
Alpha Helices
Alpha helices are one of the primary structural motifs found in proteins. These right-handed coils occur when hydrogen bonds form between the backbone atoms in the peptide chain, pulling it into a helical shape. This feature is significant because it contributes to the overall stability of the enzyme.
Their compact structure allows enzymes to fold efficiently, minimizing the energy required for conformational stability. The presence of alpha helices adds versatility to enzymes, as their arrangement can lead to functional sites that play crucial roles in catalytic activity. However, too many helices can lead to rigidity that might limit substrate binding.
Beta Sheets
Beta sheets are another essential component of secondary structure. Composed of two or more strands lined up side by side, they form strong, stable structures held together by hydrogen bonds, which provides a sort of foundation for the enzyme's shape. Their unique ability to create a flat, sheet-like appearance allows them to form complex interactions within the enzyme.
The key characteristic of beta sheets lies in their foundational stability, making them well-suited for many enzyme functions. However, while their stability is a strong point, if overly relied upon, the lack of flexibility might hinder certain enzymatic reactions that require fluid conformational adjustments.
Tertiary Structure: 3D Configuration
Domains
Domains are distinct structural or functional units within a protein. They can carry out particular functions independently, making them essential for overall enzyme activity. This modular nature allows for the evolution of proteins through the rearranging of domains, which can lead to new functionalities.
The molecular diversity seen in enzymes can often be attributed to these domains, making them integral to enzyme functionality. Their specific arrangements can also dictate the spatial configuration necessary for binding substrates or other cofactors. Still, misfolding of domains can lead to loss of function, demonstrating their critical role in enzymatic pathways.
Active Sites
Active sites are the heart of any enzyme, where substrates bind and reactions occur. The specific arrangement of amino acids forms a unique pocket that allows for the selective binding of substrates, driving the catalytic process. Active sites are fundamental to enzyme specificity, delivering their capability to carry out precise reactions.
The importance of active sites cannot be overstated, as they determine how well an enzyme performs its job. Their unique configurations allow them to distinguish between similar substrates—however, this specificity can be a double-edged sword; while it makes the enzyme efficient, it also means that a change in the structure could potentially render the enzyme inactive.
Quaternary Structure: Multi-Subunit Complexes
Homomeric and Heteromeric Enzymes
Homomeric enzymes consist of identical subunits, while heteromeric enzymes are made up of different types. This structural variety is a key aspect of enzymatic functionality, influencing how they interact with substrates, cofactors, and other molecules in their environment. Their quaternary structure often enhances catalytic efficiency and regulation.
Homomeric enzymes can capitalize on their symmetry, which often results in simpler control mechanisms during enzymatic reactions. On the other hand, heteromeric enzymes can achieve higher levels of complexity and functional diversity, allowing these enzymes to play distinct roles in various biochemical pathways. However, the added complexity might result in challenges for larger systems when analyzing their behavior.
Examples of Quaternary Structures
Examining examples of quaternary structures reveals the impressive range of enzyme complexity. Hemoglobin and DNA polymerase, for instance, showcase the importance of subunit interactions for functionality. Observing such structures emphasizes how critical the collaborative behavior of enzyme subunits is for overall catalytic efficacy.
This multi-subunit organization can provide both advantages in versatility and challenges in maintaining stability and coordination through various cellular conditions.
In summary, understanding the molecular constitution of enzymes provides essential insights into their function. Each structural level contributes uniquely to enzyme activity, thereby underlining their significance in both biological processes and biotechnological applications.
Cofactors and Coenzymes
Cofactors and coenzymes are critical components that contribute significantly to the functionality and efficiency of enzymes. They are like a well-tailored suit that fits just right, enhancing the overall working performance of their respective enzymes. The discussion around these molecules is not only pivotal to understanding how enzymes operate but also sheds light on their broader implications in biochemical processes and biotechnological applications.
Definition and Importance
In the simplest terms, cofactors are non-protein chemical compounds that assist enzymes during the catalysis of reactions. They are essential for the enzyme’s biological activity. Without them, enzymes can be rendered inactive, somewhat like a musician without an instrument. Coenzymes, on the other hand, are organic molecules that serve as cofactors and often act as carriers of electrons, atoms, or functional groups that are transferred in biochemical reactions. Their role cannot be understated—these molecules are often the difference between an enzyme that works effectively and one that doesn’t. Enzymes and their cofactors together create a dynamic and responsive system that drives countless biological processes.
Types of Cofactors
Cofactors can be broadly categorized into inorganic and organic types, each with unique characteristics and functions.
Inorganic Cofactors
Inorganic cofactors are typically metal ions such as zinc, iron, and magnesium. They play vital roles in stabilizing enzyme structures and participating directly in the catalytic process. For instance, zinc is crucial for the activity of certain proteases, as it helps maintain proper enzyme conformation, allowing the active site to bind substrates effectively. One key characteristic of inorganic cofactors is their ability to provide electrostatic interaction and facilitate substrate orientation. This makes them not only beneficial but also indispensable in numerous enzyme-catalyzed reactions.
The major appeal of inorganic cofactors lies in their varied roles across different enzyme types, enhancing specificity and efficiency. However, their presence can also bring challenges; some enzymes may become dependent on these metal ions, and any deficiency can lead to diminished enzyme activity, causing adverse physiological effects.
Organic Cofactors
Organic cofactors, or coenzymes, are often derived from vitamins. A classic example is NAD+ (nicotinamide adenine dinucleotide), a critical coenzyme in redox reactions. The advantageous aspect of organic cofactors is their ability to engage in a variety of chemical reactions, often serving as intermediate carriers in metabolic pathways. For example, coenzyme A is vital in fatty acid metabolism and the Krebs cycle, acting as a carrier for acetyl groups.
Their unique feature is their dynamic structure, which often allows them to participate directly in enzymatic reactions by donating or accepting chemical groups. This versatility is what makes organic cofactors popular choices in both enzymatic processes and biotechnological applications. Nonetheless, the dependence on specific organic cofactors can sometimes lead to vulnerabilities, particularly when it comes to dietary insufficiencies affecting enzyme activity.
Mechanism of Action
Understanding the mechanisms through which cofactors and coenzymes operate is crucial for grasping their broader role in enzymatic function.
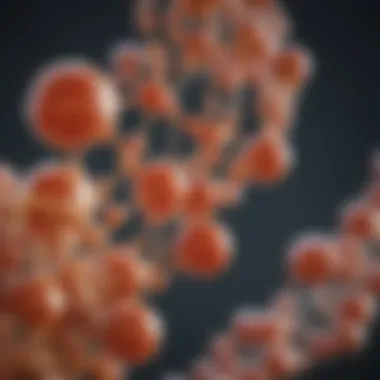
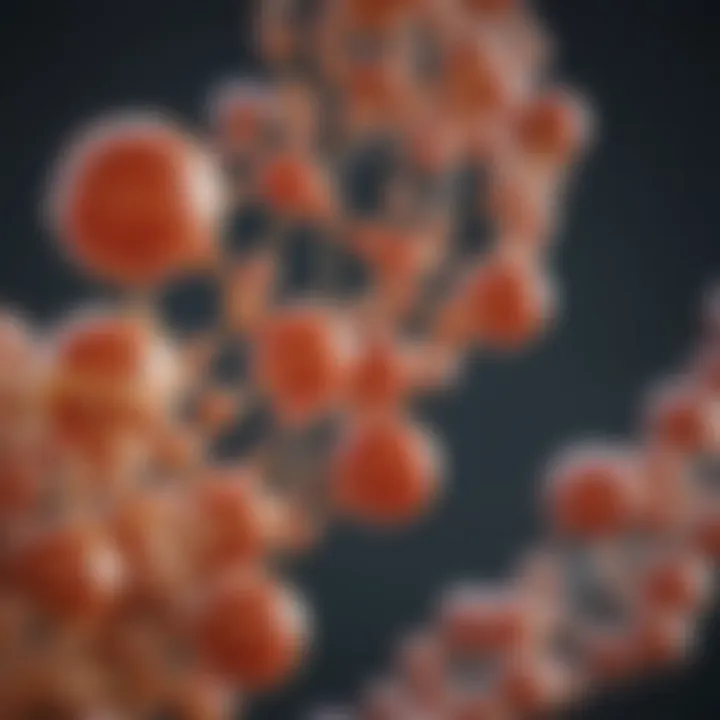
Activation of Enzyme Activity
Activation of enzyme activity is often facilitated by the binding of a cofactor or coenzyme, which induces a conformational change in the enzyme. This adaptation is essential for the enzyme to acquire its active form, increasing its efficiency and throughput. Activating enzymes can almost be thought of as flipping a switch to turn on a machine—without that spark, the reaction simply doesn’t happen. The introduction of coenzymes has been shown to enhance the rates of many biochemical reactions drastically, emphasizing their importance in metabolic pathways.
Role in Catalysis
In terms of catalysis, cofactors play vital roles in the transformation of substrates into products. They can assist in stabilizing transition states or providing necessary chemical groups for the reaction to proceed. For example, certain cofactors can alter the electrostatic environment around the active site, enhancing substrate binding and making the catalysis more efficient. This characteristic is beneficial because it opens up possibilities for the development of enzyme inhibitors or drugs that specifically target the cofactor-enzyme interaction, providing a platform for therapeutic advancements.
The cofactor can be likened to a conductor in an orchestra, ensuring that every section plays in harmony to achieve timely and accurate execution of a symphony.
Factors Affecting Enzyme Activity
Enzyme activity can be influenced by several factors that are crucial to their performance in catalyzing biochemical reactions. Understanding these factors is key for researchers and practitioners, particularly in fields like biotechnology and pharmaceuticals, where enzymes play a vital role in various processes. A comprehensive grasp of the conditions that can enhance or inhibit enzyme action not only aids in developing robust applications but also informs safe practices regarding enzyme use in different environments.
Temperature and pH
Optimal Conditions
The concept of optimal conditions serves as a guiding principle in enzyme functionality. Each enzyme has a specific temperature and pH range in which it operates best. For most enzymes, a temperature around 37°C—akin to human body temperature—proves favorable. This characteristic is especially beneficial because, at this temperature, enzyme reactions can reach their peak rates without significant risk of damaging the active site or structure.
However, these conditions vary among enzymes; some may thrive in more extreme environments, as seen in extremophiles. The unique feature of this variability is its significance in evolutionary biology. It showcases how enzymes adapt to their specific ecological niches. The main advantage of understanding these optimal conditions is the enhancement of enzyme efficiency, which is particularly pertinent in industrial processes where even minor improvements can lead to significant cost savings.
Denaturation
Denaturation is a phenomenon that can drastically affect enzyme functionality. When an enzyme is subjected to extreme temperatures or unfavorable pH levels, its structure can become altered—this process is what we call denaturation. Once an enzyme denatures, it loses its active site configuration, rendering it incapable of binding with substrates. The critical aspect here is that denaturation is often irreversible, which underlines the importance of maintaining optimal conditions during enzymatic reactions.
While the practicality of denaturation may seem to present limitations, it also showcases the significance of enzyme stability for applications. For instance, enzymes in pharmaceuticals must withstand various conditions to maintain efficacy. The unique feature of understanding denaturation lies in its implications for the design of more resilient enzymes that can endure harsher environments.
Substrate Concentration
Michaelis-Menten Kinetics
Michaelis-Menten kinetics describes how enzyme reactions respond to changes in substrate concentration. When substrate is plentiful, the rate of reaction increases until it reaches a saturation point, only to slow as available enzyme molecules become fully engaged. This kinetics model is essential because it provides a mathematical framework for predicting enzyme activity based on substrate levels.
The significant characteristic of this model is its applicability across various biological contexts, rendering it a beneficial choice for this article. Its advantages include offering insights into enzyme efficiency and potential bottlenecks in metabolic pathways. However, it is important to recognize its limitations; it assumes a single substrate, which may not capture the complexities of multi-substrate reactions in living organisms.
Saturation of Enzyme
The saturation of enzyme is a fundamental concept indicative of the point at which all available enzyme active sites are occupied by substrates. This state leads to a plateau in reaction rates, signifying maximum velocity. Understanding saturation is vital, as it gives insight into the efficient functioning of enzymes in various biological scenarios.
One key advantage is its implication in drug development, where determining optimal enzyme saturation can maximize therapeutic efficacy while minimizing side effects. However, this saturation doesn't mean enzymes are inactive beyond this point; instead, it highlights the importance of substrate availability for sustained reaction rates.
Inhibitors: Competitors and Non-competitors
Inhibitors play a crucial role in enzyme activity, with the potential to significantly slow down or completely halt enzymatic reactions. They can be classified primarily into competitive and non-competitive inhibitors. Competitors typically vie for the same active site as the substrate, decreasing reaction rates by limiting available substrate for binding. Essentially, they hold enzyme behaviors at bay. On the other hand, non-competitive inhibitors bind to different parts of the enzyme, modifying its structure and usability—even if the substrate is present.
The understanding of these inhibitors is key for both academic and practical applications, particularly in developing treatments for diseases where enzyme activity needs regulation. The implications of using inhibitors in clinical settings are profound, providing a powerful toolkit for managing metabolic pathways.
Enzyme Specificity
Enzyme specificity is a pivotal concept in biochemistry, referring to how enzymes are selective for particular substrates during reactions. This selectivity is not merely a trivial detail; it underpins the precision with which enzymes function in metabolic pathways. The ability of an enzyme to act on a specific substrate or a limited range of substrates is crucial for maintaining the delicate balance of biochemical processes in living organisms. In this article, we will delve into two primary models that explain enzyme specificity: the Lock and Key model and the Induced Fit model. Exploring these models not only provides insight into how enzymes operate, but it also helps in understanding their roles in diverse biological functions.
Lock and Key Model
The Lock and Key model, introduced by Emil Fischer in the early 20th century, presents a straightforward analogy: enzymes are like keys, and substrates are like locks. This model posits that the enzyme’s active site, which is a specific region on the enzyme, has a unique shape complementary to that of its substrate. Just as a key fits into a lock, only the right substrate can bind to the active site of the enzyme.
This specificity ensures that enzymes catalyze only specific reactions, thereby preventing unwanted or dangerous biochemical activities. The simplicity of the Lock and Key model helps explain the manner in which enzymes exhibit selective catalysis. However, it does not account for the dynamic nature of enzymes, as they can adapt slightly to accommodate their substrates. This leads us into the next concept.
Induced Fit Model
In sharp contrast to the Lock and Key theory, the Induced Fit model offers a more nuanced perspective. Proposed by Daniel Koshland in 1958, this model suggests that the binding of a substrate induces a change in the shape of the enzyme's active site. This alteration enhances the fit between enzyme and substrate, akin to a flexible key that molds itself to the lock upon entry.
This flexible approach explains how enzymes can maintain their specificity while still being adaptable. It further illuminates how enzymes can catalyze reactions by lowering the activation energy more effectively when the substrate is positioned precisely in the active site, facilitated by these structural changes. The Induced Fit model emphasizes the importance of conformational flexibility, which is vital for enzyme functionality.
"Enzyme specificity is the reason why biochemical reactions can be finely tuned and regulated to ensure life processes proceed correctly."
In summary, enzyme specificity is a central theme in understanding how enzymes function. It highlights the precision of biochemical interactions, ensuring that enzymes are tailored for their specific substrates, whether one thinks in terms of fixed shapes like the Lock and Key or dynamic adaptations as proposed by the Induced Fit model. This specificity has vast implications in biotechnology, pharmaceuticals, and understanding metabolic pathways, paving the way for targeted therapies and enzyme engineering.
Enzyme Evolution and Adaptation
The study of enzyme evolution and adaptation provides deep insights into how these vital proteins have changed over time. Understanding this framework is essential to appreciate the complexity of biochemical processes. Enzymes evolve through natural selection, adapting to meet the demands of their environment. This adaptive process not only enhances their efficiency but also broadens their functional repertoire, which significantly affects various biological systems. It’s fascinating how a simple change in an amino acid can transform an enzyme's capability, reflecting the intricate relationship between structure and function.
Natural Selection of Enzyme Functions
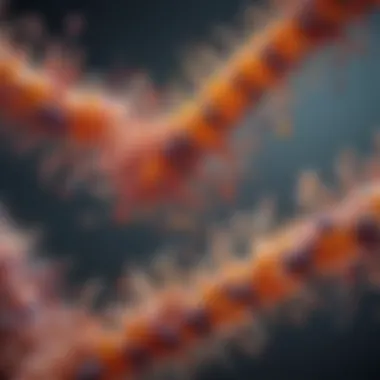
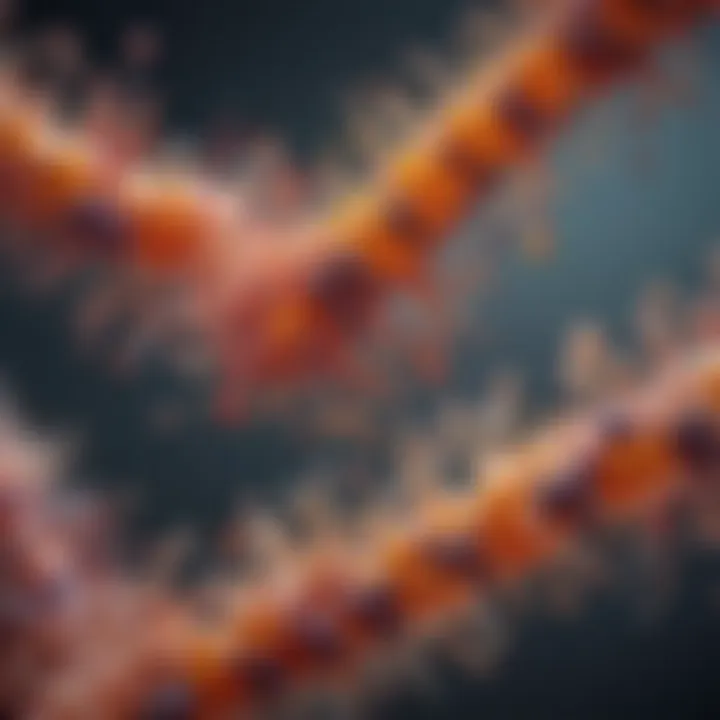
Natural selection plays a pivotal role in determining which enzymes will thrive. Just think about it—an enzyme that can facilitate a reaction more efficiently will be favored by nature over its less effective counterparts. Over time, specific functions become embedded in their sequences, allowing organisms to adapt to varying environmental pressures.
- Survival of the Fittest: Only those enzymes that provide an advantage in metabolic pathways survive. For instance, in extreme environments like hot springs, enzymes that maintain their structure at high temperatures are selected.
- Diversity: Enzymes are not one-size-fits-all; they evolve to accommodate different substrates, leading to a stunning variety in enzyme functionality across species.
This evolutionary aspect of enzyme adaptation not only showcases the brilliance of nature but also sparks interest in how understanding these processes can aid technological advancements.
Enzyme Engineering
The field of enzyme engineering is all about harnessing this evolutionary knowledge to create tailored enzymes that meet specific needs, especially in biotechnology. There are two predominant methods in this area: directed evolution and rational design. Each has its own favorable traits and applications, making them crucial tools in the enzyme engineering toolbox.
Directed Evolution
Directed evolution mimics natural selection in a lab setting. By introducing mutations randomly to an enzyme, scientists create a vast library of variants. The essence here is to screen these variants under selective conditions to find those that outperform.
- Key Characteristic: This technique often leads to enzymes with capabilities far beyond what nature can provide.
- Beneficial Choice: It is widely popular due to its effectiveness in producing enzymes optimized for industry applications, like biodegradable plastics or biofuels.
- Unique Feature: One notable strength is the ability to incorporate a wide range of mutations, resulting in diverse catalytic properties.
- Advantages and Disadvantages: While directed evolution yields novel enzymes, it can be resource-intensive and time-consuming. There’s also the risk of unintended consequences if an enzyme behaves unpredictably.
Rational Design
On the other hand, rational design employs a more calculated approach. It relies on understanding an enzyme's structure and function down to the atomic level. Enzyme models are used to predict how changes in the amino acid sequence will impact performance.
- Key Characteristic: It allows for targeted modifications, aiming for specific attributes or functions within the enzyme.
- Beneficial Choice: This approach is especially valuable in pharmaceutical applications, where precision is crucial.
- Unique Feature: Rational design enables the prediction of enzyme behavior before synthesis, which can save time and resources.
- Advantages and Disadvantages: However, its reliance on prior structural knowledge limits its applicability to well-studied enzymes, leaving less room for improvisation. Additionally, the accuracy of predictions can vary, which may lead to trial and error in enzyme development.
In sum, the evolution and engineering of enzymes open doors to a plethora of possibilities. Innovations in these areas stand at the forefront of biotechnological advancements, impacting fields ranging from medicine to industrial processes.
Applications of Enzymes in Biotechnology
Enzymes serve as the unsung heroes in the realm of biotechnology, bridging the gap between biology and technology with their remarkable ability to catalyze biochemical reactions. Their diverse applications underscore their significance, not just in nature but also in various scientific and industrial fields. By harnessing these biological catalysts, researchers and manufacturers have unlocked potential across multiple sectors, propelling advancements that benefit society in substantial ways.
Pharmaceutical Development
In the pharmaceutical industry, enzymes are instrumental in drug design and production. They enable more precise chemical reactions and help synthesize complex molecules efficiently. One crucial aspect of using enzymes in this sector is their specificity; many enzymes act only on certain substrates, which ensures that unwanted by-products are minimized. This characteristic is especially vital when creating drugs that require a high level of purity and consistency.
- Efficiency: Enzymes can often catalyze reactions at much lower temperatures and pressures than traditional chemical processes. This not only saves energy but also helps maintain the integrity of sensitive compounds.
- Green Chemistry: The use of enzymes in drug synthesis aligns with green chemistry principles by reducing the reliance on hazardous chemicals and solvents.
Given the intricate nature of human biochemistry, enzyme-based innovations have unlocked doors to new therapies for diseases that were once considered challenging or impossible to treat.
Industrial Applications
The industrial sector capitalizes on enzymatic reactions for their inherent advantages, which range from superior efficiency to environmental friendliness. Enzymes usher in a new era where traditional methods are often overshadowed by biocatalysis, presenting a more sustainable and cost-effective alternative.
Biocatalysis
Biocatalysis refers to the use of natural catalysts, such as enzymes, to facilitate chemical transformations in synthetic processes. One specific aspect of biocatalysis that contributes to its growing popularity is its ability to catalyze reactions under mild conditions. This characteristic is crucial, as it reduces the energy input, making the process not just efficient but also less harmful to the environment.
- Key Characteristics: Among its numerous traits, the selectivity of biocatalysis stands out, allowing for the desired product to be formed without the formation of unwanted by-products. This specificity results in cleaner processes that are both economical and environmentally friendly.
- Unique Feature: Additionally, biocatalysis can operate in diverse conditions, such as varying pH and temperatures, which makes it highly adaptable to different industrial needs.
The benefits of integrating biocatalysis into industrial applications are numerous, including reduced waste, lower energy consumption, and enhanced product yields.
Bioremediation
Bioremediation, another vital application of enzymes, focuses on using microorganisms and enzymes to clean up contaminated environments, such as soil and water. This process capitalizes on the natural ability of certain enzymes to break down pollutants, making them less harmful or even harmless.
- Key Characteristics: One main characteristic of bioremediation is its effectiveness in treating hazardous waste and restoring ecosystems affected by pollutants, such as heavy metals or oil spills. This eco-friendly approach to waste management is gaining momentum as the world seeks sustainable solutions to environmental challenges.
- Unique Feature: The adaptability of bioremediation means it can be tailored to suit various contaminants, making it a versatile option for environmental restoration efforts.
The advantages of this application cannot be overstated, offering not only a cost-effective solution for cleanup operations but also promoting the recovery of natural habitats.
Enzymes, with their unparalleled specificity and efficiency, have paved the way for innovative applications across biotechnology, reshaping industries and enhancing sustainability through various processes.
Closure
The conclusion serves as a pivotal point in this discussion about enzymes, rounding out the intricate details explored throughout the article. By reiterating key insights into enzyme composition and functionality, it highlights their significance not just in biological processes, but also in practical applications across various fields. This recap is essential; it consolidates the foundational knowledge needed for students, researchers, and professionals alike to navigate the complex biochemistry involving enzymes.
Enzymes are not merely passive actors in biochemical reactions. They are dynamic catalysts, essential for sustaining life and driving metabolic pathways. Their composition—ranging from amino acids to cofactors—dictates their activity, specificity, and efficiency. A comprehensive understanding of these aspects provides a clearer picture of how biological systems operate and the potential they hold for industrial and pharmaceutical advancements.
"Understanding enzymes is key to unlocking innovations in medicine, agriculture, and environmental science."
By mastering this foundational topic, researchers are better equipped to harness the unique properties of enzymes for innovative solutions, like designing new drugs or developing more efficient industrial processes. As the fields of biotechnology and enzyme engineering evolve, the practical implications become even more pronounced, emphasizing the need for ongoing research and education.
Summary of Key Points
To encapsulate the most salient points from our exploration:
- Enzymes act as catalysts, accelerating biochemical reactions crucial for life.
- Their structures—primary through quaternary—determine their functionality, with different levels of organization contributing to active site formation and overall activity.
- Cofactors and coenzymes play essential roles in enhancing enzyme performance, showcasing the collaborative nature of these biomolecules.
- Factors such as temperature, pH, and substrate concentration significantly influence enzyme kinetics and effectiveness.
- Understanding enzyme specificity and adaptation highlights their importance in evolutionary terms and practical applications.
- Biotechnological applications range from drug development to industrial processes, underpinning their relevance beyond academic inquiry.
Future Directions in Enzyme Research
As we look ahead, several avenues appear ripe for further exploration in enzyme research:
- Directed Evolution: Tailoring enzymes through synthetic evolution techniques could result in custom-made catalysts with enhanced specificity or activity, providing solutions to some of today’s pressing challenges in various sectors.
- Rational Design: Leveraging advanced computational tools and structural biology to predict enzyme behavior allows for a more targeted approach in designing enzymes for specific tasks.
- Environmental Applications: Enzymes have the potential to help in bioremediation efforts, allowing for better degradation of pollutants and waste products, making them invaluable for environmental restoration projects.
- Pharmaceutical Innovation: Studying enzyme mechanisms could lead to breakthroughs in drug design, targeting specific pathways in disease processes and resulting in more effective treatments with fewer side effects.
In summary, enzyme research is poised to continue its trajectory of innovation and importance. As scientific techniques advance and the need grows for sustainable solutions in a rapidly changing world, a thorough understanding of enzymes will be indispensable.