Exploring Oligonucleotide Secondary Structures
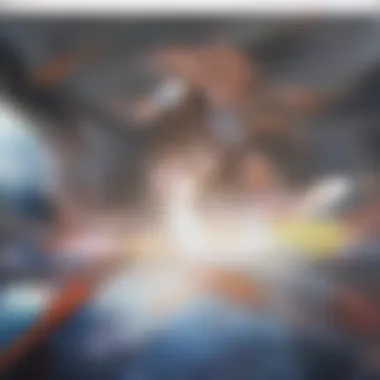
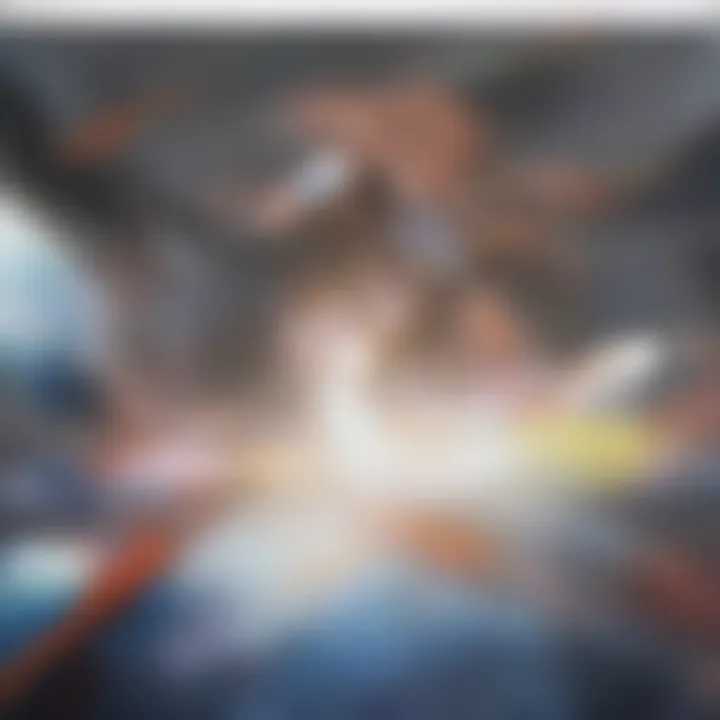
Intro
Oligonucleotides are short sequences of nucleic acids that play critical roles in various biological systems. Their secondary structures can influence their stability, functionality, and interaction with other molecules. Understanding these structures is essential for many applications in genetic research and therapeutic development. This article will analyze the significance of oligonucleotide secondary structures, exploring the principles that govern them as well as the technologies employed to study these conformations.
Research Methodology
Description of Research Design and Approach
The research design chosen for studying oligonucleotide secondary structures integrates both theoretical and empirical methods. Theoretical models provide insights into how these structures can form, whereas empirical methods involve laboratory techniques to validate these models. The combination of both approaches allows a comprehensive understanding of oligonucleotide dynamics and interactions.
Materials and Methods Used in the Study
Key materials employed in the research include:
- Synthetic oligonucleotides, sourced from companies like Integrated DNA Technologies.
- Buffers and reagents for biochemical assays.
- Instruments like circular dichroism (CD) spectropolarimeter and dynamic light scattering (DLS) setups for characterizing the structures.
The methods employed for analysis often include:
- NMR spectroscopy for determining detailed structural information.
- Gel electrophoresis for assessing structural stability and interactions under various conditions.
- Computational simulations to predict structural conformations based on thermodynamic principles.
Discussion and Interpretation
Interpretation of Results in the Context of Existing Literature
The research findings reflect a progressive understanding of oligonucleotide secondary structures. For example, the stability of certain motifs, such as hairpins or loops, was confirmed by both experimental and computational methods. This correlation with existing literature supports the validity of the approaches used in the study and opens avenues for further exploration.
Implications for Future Research or Practical Applications
The future of genetic research heavily relies on our capacity to manipulate oligonucleotide structures effectively.
Intro to Oligonucleotides
Oligonucleotides are short chains of nucleotides linked together by phosphodiester bonds. Their unique properties make them significant in various fields of biological research and application. They serve as the fundamental units in DNA and RNA, the molecules that carry genetic information. This introductory section aims to highlight the importance of understanding oligonucleotides in the broader context of genetic research and therapeutic development.
The exploration of oligonucleotides is crucial for several reasons. First, they play a key role in molecular biology techniques such as PCR (Polymerase Chain Reaction), sequencing, and gene editing. Knowledge of oligonucleotide types and functions contributes to advancements in these areas, promoting innovation in genetic engineering and synthetic biology. Furthermore, oligonucleotides are used extensively in diagnostics and therapeutics, emphasizing their practical applications in medicine.
Definition of Oligonucleotides
Oligonucleotides are polymers composed of about 2 to 200 nucleotides, which can be either DNA or RNA. Their sequences can be designed to target specific nucleic acid sequences in various biological processes. By crafting specific sequences, researchers manipulate gene expression or silence particular genes. Thus, oligonucleotides serve as tools that are invaluable for both understanding genetic functions and developing new therapeutic strategies.
Types of Oligonucleotides
Oligonucleotides can broadly be categorized into two main types: DNA oligonucleotides and RNA oligonucleotides. Understanding these distinctions is essential for appreciating their unique roles in research and applications.
DNA Oligonucleotides
DNA oligonucleotides are composed solely of deoxyribonucleic acid. Their key characteristic is their stability in various environments. This stability allows them to effectively bind to complementary DNA sequences. Because they are more resistant to degradation, DNA oligonucleotides are a popular choice in numerous applications, including gene synthesis and amplicon generation.
One unique feature of DNA oligonucleotides is their use in the design of primers for PCR. These primers are crucial for amplifying specific DNA segments, which is essential for various genetic analyses. A notable advantage of DNA oligonucleotides in research is their predictability in hybridization due to Anfinsen's principles of nucleic acid folding. However, their stability can also pose challenges in certain experimental designs, as unwanted secondary structures might form, potentially interfering with results.
RNA Oligonucleotides
RNA oligonucleotides contain ribonucleic acid, which gives them different properties compared to their DNA counterparts. A key characteristic of RNA oligonucleotides is their ability to form intricate secondary structures due to the presence of a hydroxyl group at the 2' position on the ribose sugar. This structural capability is beneficial for various biological functions, including regulatory processes in gene expression.
Moreover, RNA oligonucleotides are used extensively in therapeutic applications such as RNA interference (RNAi) and antisense strategies. A unique feature of RNA oligonucleotides is their susceptibility to nucleases, which can be seen as both an advantage—in terms of rapid metabolism in living cells—and a disadvantage, complicating long-term applications. Understanding these oligonucleotide types leads to better decision-making in experimental design and therapeutic strategy development.
The Basics of Secondary Structures
Understanding secondary structures is crucial to appreciating the role of oligonucleotides in biological systems. These structures provide essential insights into the stability and functionality of nucleic acids. By examining these configurations, one can glean information about how oligonucleotides interact within cellular environments, how they fold, and their overall behavior in various biological processes. The study of secondary structures gives context for the predictive models and analytical techniques that follow throughout this field.
Importance of Secondary Structure
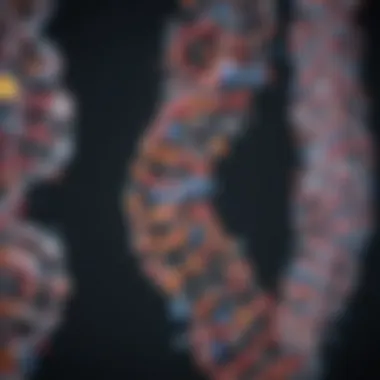
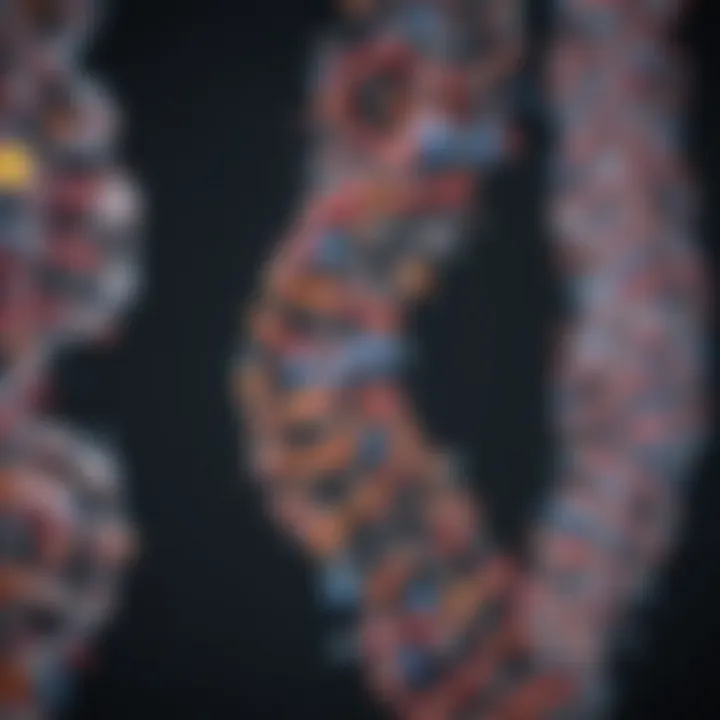
Secondary structures play a fundamental role in the functionality of nucleic acids. Oligonucleotides can form distinct arrangements such as hairpins, loops, and bulges, which may influence their biological activity. These forms are not simply random; rather, their specific shapes often determine how these molecules will interact with proteins and other nucleic acids. For example, an oligonucleotide's secondary structure can enhance or hinder its ability to bind to target sequences, thereby impacting gene expression or the efficacy of drugs. Therefore, understanding these structures is not only an academic consideration but also has practical implications in genetics and therapeutic development.
Factors Influencing Oligo Secondary Structures
Several factors contribute to the formation of secondary structures in oligonucleotides. Understanding these elements is key for predicting how an oligonucleotide will behave under different conditions. The main factors include:
Hydrogen Bonding
Hydrogen bonding is a critical interaction in the formation of secondary structures. These bonds can occur between complementary bases, such as adenine pairing with thymine, enabling the formation of double helical structures. The key characteristic of hydrogen bonding is its specificity; only certain bases can pair correctly, which is crucial for accurate base pairing and thus proper folding. This feature makes hydrogen bonding a beneficial choice for maintaining the structural stability of an oligonucleotide. As a result, the unique ability of hydrogen bonding to create distinct structural formations helps oligonucleotides perform their roles effectively in biological systems. However, this dependence on specific sequences can be a limitation when unintended pairings occur, potentially disrupting function.
Base Stacking
Base stacking is another important factor in stabilizing secondary structures. This phenomenon happens when non-covalent forces, such as van der Waals forces, draw adjacent bases closer together in a stacked configuration. The primary characteristic of base stacking is its contribution to the overall stability of nucleic acid structures. This feature is vital for the formation of helical structures, providing both mechanical strength and stability to the oligonucleotide. The unique advantage of base stacking is that it facilitates the overall compactness of the molecule, allowing for more efficient folding. However, if there is inadequate stacking, it can lead to destabilization, ultimately affecting the oligonucleotide's function.
Ion Presence
The presence of ions in the environment also significantly influences oligonucleotide secondary structures. Ions, particularly metal ions like magnesium and sodium, stabilize the structure by neutralizing the negative charges on the phosphate backbone, thereby reducing repulsive forces. The key characteristic of ion presence is its role in maintaining the structural integrity of oligonucleotides. This feature is crucial for functions like enzymatic activity and binding interactions. While the effect of ions is predominantly stabilizing, excessive concentrations can lead to changes in structural dynamics, potentially altering biological function.
Understanding these factors offers a comprehensive view of how oligonucleotides attain their shapes and maintain their stability.
In summary, delving into the basics of secondary structures unveils essential insights into the functionality of oligonucleotides. Through hydrogen bonding, base stacking, and ion presence, these molecules can achieve specific formations that have significant implications in biochemical processes.
Key Concepts in Secondary Structure Formation
Understanding the formation of secondary structures in oligonucleotides is crucial. These concepts help elucidate how nucleotide sequences fold and interact, impacting their biological functions and applications. The structure of oligonucleotides plays a significant role in processes such as gene regulation and molecular recognition. Key aspects include thermodynamic stability and molecular interactions, which dictate how these structures form and persist in biological environments.
Thermodynamic Stability
Thermodynamic stability is essential for the formation of secondary structures. It refers to the energy balance that dictates whether a particular structure is favored or not in a given environment. A stable structure will have lower free energy, making it less likely to unfold. Several factors contribute to this stability:
- Hydrogen Bonds: Interactions between complementary bases provide significant stabilization.
- Base Stacking: Adjacent bases stack on top of each other, minimizing exposure to water, which is energetically favorable.
- Ionic Strength: Metal ions can stabilize the structure by neutralizing negative charges on the phosphate backbone.
Therefore, understanding thermodynamic stability aids in predicting which secondary structures are feasible under specific conditions in experiments or therapeutic scenarios.
Molecular Interactions
Molecular interactions are integral to the formation of oligonucleotide secondary structures. These interactions can be categorized into intramolecular and intermolecular types.
Intramolecular vs. Intermolecular
Intramolecular interactions occur within the same oligonucleotide strand, allowing regions of the sequence to fold back and base pair with themselves. This self-complimentary nature is an important characteristic, particularly in the formation of structures like hairpins or loops.
On the other hand, intermolecular interactions involve different strands. They can form complexes, such as duplexes, where complementary sequences bind together. These types of interactions expand the functional diversity of oligonucleotides, influencing areas like gene silencing and therapeutic design. Intramolecular interactions tend to be more stable in certain contexts, while intermolecular interactions facilitate broader applications in gene regulation and diagnostics.
"Understanding the balance of intramolecular and intermolecular interactions is critical for leveraging oligonucleotides in therapeutic development."
Specific vs. Non-Specific Interactions
Specific interactions occur when particular nucleotide sequences recognize and bind to their complementary partners with high affinity. This specificity is crucial for processes such as DNA replication where precision is vital.
Non-specific interactions happen between oligonucleotides that do not rely on complementary base pairing. These interactions can lead to aggregation or interference, which might pose challenges or opportunities in experimentation. For instance, non-specific interactions can complicate the interpretation of results in binding studies.
Both types of interactions have distinct benefits. Specific interactions provide targeted mechanisms in gene therapy, while non-specific interactions can allow for broader investigations into oligonucleotide behavior in complex biological systems. However, understanding the implications of both interactions can clarify how oligonucleotide secondary structures function and their potential applications.
Techniques to Analyze Oligo Secondary Structures
Analyzing oligonucleotide secondary structures is crucial in understanding their biological roles and potential applications. These techniques enable researchers to elucidate structural information that is vital for predicting function, stability, and interactions with other molecules. Some significant elements associated with these methods include accurate information retrieval, enhanced predictive models, and insights into molecular behaviors. Understanding these techniques also emphasizes the need for careful consideration, particularly regarding the conditions under which experiments are conducted, as various factors can affect outcomes.
Nuclear Magnetic Resonance (NMR) Spectroscopy
Nuclear Magnetic Resonance (NMR) Spectroscopy is a powerful tool for studying oligonucleotide structures in solution. This technique provides detailed information about the conformational states of oligonucleotides, allowing researchers to observe dynamic movements within the molecule. NMR is particularly useful because it can yield data about specific atom interactions without needing crystallization, which is often a requirement for other methods.
The primary advantage of NMR is its ability to provide real-time structural data, important for understanding how oligonucleotides behave in biological systems. However, there are considerations to keep in mind. Factors such as concentration, temperature, and ionic conditions can significantly impact the resulting data, leading to differing interpretations. Therefore, consistent and carefully controlled experimental conditions are paramount to obtaining reliable results.
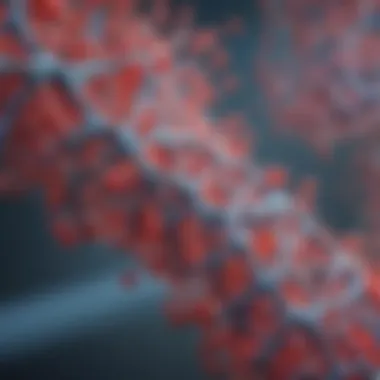
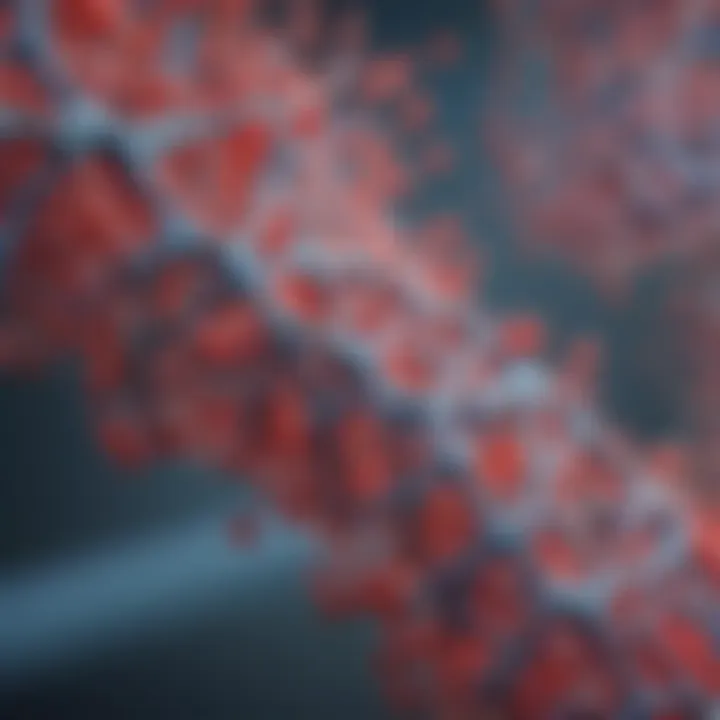
X-ray Crystallography
X-ray crystallography stands as another fundamental technique for analyzing oligonucleotide secondary structures. This method requires the formation of high-quality crystals, which can be challenging for oligonucleotides due to their inherent flexibility and tendency to form diverse structures. However, once successfully crystallized, X-ray crystallography can offer high-resolution images of the molecular structure, revealing intricate details about molecular arrangement.
The strength of X-ray crystallography lies in its ability to generate precise three-dimensional structures. While this level of detail is invaluable, the process can be time-consuming and often requires considerable optimization. In addition, the static nature of crystallized structures may not always represent the dynamic behavior of oligonucleotides in solution, which could lead to discrepancies in understanding their real-world applications.
Computational Modeling
Computational modeling has emerged as a significant method for predicting oligonucleotide secondary structures. This approach utilizes algorithms and software to simulate various folding pathways and predict thermodynamic stability. Tools like Mfold and RNAfold have gained popularity due to their utility in generating probable secondary structures based on given sequences.
While computational modeling offers a rapid way to assess potential structures, some limitations exist. The accuracy of these predictions often hinges on the quality of the algorithms and the underlying data used in the models. Therefore, validation against experimental data remains crucial. Additionally, the computational resources required for these simulations can be considerable, affecting accessibility for some researchers.
Predicting Oligonucleotide Secondary Structures
Predicting oligonucleotide secondary structures is crucial for understanding their function in biological systems. These predictions help to elucidate how the sequences fold and how they interact with other molecular entities. With applications spanning from gene regulation to therapeutic design, a robust model for prediction can vastly improve both comprehension and application.
The methods developed for these predictions leverage complex mathematical algorithms and software tools. The accurate modeling of secondary structures can assist researchers in identifying binding sites and understanding the stability of specific structures. This is not just useful for academics; it impacts clinical settings where oligonucleotides are used as therapeutic agents.
Algorithms and Software
Mfold
Mfold is one of the widely used software tools for predicting the secondary structures of nucleic acids. Its key characteristic lies in its ability to predict the most thermodynamically stable structures from a given sequence. Mfold stands out due to its user-friendly interface, allowing researchers to input sequences easily and obtain results quickly.
A significant aspect of Mfold is its unique feature of accommodating various environmental conditions, such as temperature and ionic concentration. This adaptability gives it an edge, especially when researchers need to simulate real physiological conditions. However, Mfold's predictions can sometimes overlook less stable structures that might play important functional roles.
RNAfold
RNAfold, another well-known software, specifically emphasizes RNA secondary structure prediction. Its renowned characteristic is the dynamic programming algorithm, which calculates optimal folding configurations efficiently. This software tool is particularly advantageous due to its incorporation of free energy parameters that account for different nucleobase interactions.
A noteworthy feature of RNAfold is its ability to predict pseudoknots, a common but complex structural feature in RNA. While this increases its utility, predicting such structures also heightens the computational demands and may lead to longer processing times compared to other models.
ViennaRNA Package
The ViennaRNA Package offers a complete suite of tools aimed at the prediction and evaluation of RNA secondary structures. Its key characteristic is its comprehensive approach, combining various algorithms to ensure versatility in structural prediction. This package is beneficial for users who need a range of analytical tools at their disposal, including RNA structure editing and visualization features.
A unique advantage of the ViennaRNA Package is its capability to handle large sequences, thus making it suitable for extensive genomic studies. However, with increased functionality comes added complexity, which can be a barrier for less experienced users.
Limitations of Prediction Models
While significant progress has been made in predicting oligonucleotide secondary structures, limitations remain inherent in these models. The predictions, though often reliable, can sometimes deviate from actual structures observed experimentally. Factors such as environmental conditions, specific modifications to oligonucleotides, and the presence of cellular contexts can affect the accuracy of predictions. In summary, while these models and software offer invaluable insights, they are best used in conjunction with empirical data for enhanced reliability.
Applications of Oligonucleotide Secondary Structures
Oligonucleotide secondary structures play a vital role in various biological processes and applications. Their capacity for specific interactions with nucleic acids underlines their importance in gene regulation, therapeutics, and diagnostics. Understanding these applications not only enhances our knowledge of molecular biology but also opens avenues for innovative approaches in medicine and research.
Gene Regulation
Gene regulation is one key area where oligonucleotide secondary structures demonstrate significant influence. These structures can modulate the expression of genes by interacting with regulatory elements in RNA. For instance, small interfering RNAs (siRNAs) adopt specific shapes that enable them to bind mRNA, leading to its degradation. This RNA interference mechanism is essential for controlling gene expression and has applications in gene therapy.
The formation of hairpin loops and other structures leads to different levels of stability and efficacy in these interactions. This relationship emphasizes the necessity for precise design of oligonucleotides to achieve desired regulatory effects. In addition, antisense oligonucleotides can be designed to target specific mRNA sequences, modifying how genes are expressed. This capability is used in therapeutic scenarios where diseases caused by overexpressed genes can be treated by silencing those genes.
Therapeutic Agents
In the realm of therapeutics, oligonucleotides stand out as promising agents for treating various diseases. Their ability to form secondary structures allows them to engage with specific molecular targets. One notable example is nucleotide-based therapies for genetic disorders, where oligonucleotides can be used to correct mutations at the genomic level, a process known as gene editing.
Moreover, reducing side effects and increasing target specificity are significant advantages that oligonucleotides offer over traditional drugs. These agents can achieve high specificity through structurally-defined binding, minimizing impacts on non-target sequences. Various classes of oligonucleotide drugs are now approved, such as mRNA vaccines that rely on specific secondary structures to elicit an immune response effectively.
Diagnostic Tools
The applications of oligonucleotide secondary structures extend into diagnostics, where they serve as essential tools in detecting nucleic acid sequences associated with diseases. Techniques such as polymerase chain reaction (PCR) and lateral flow assays leverage oligonucleotide structures to identify pathogens or genetic mutations swiftly. Customized probes designed to hybridize to specific targets based on their secondary structure can enhance the sensitivity and accuracy of these diagnostic methods.
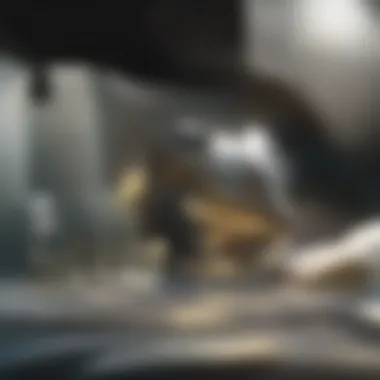
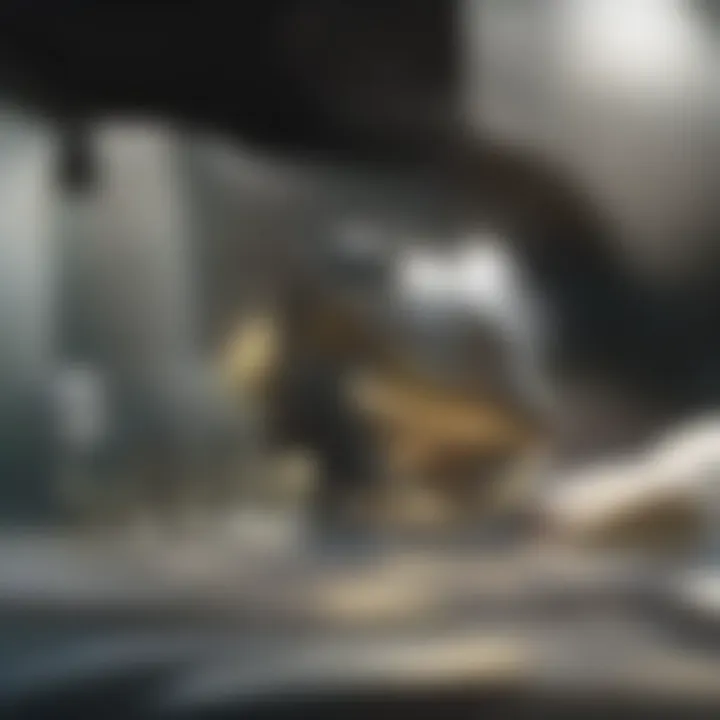
Furthermore, high-throughput sequencing technologies utilize oligonucleotide secondary structures to provide in-depth insights into complex genomes, facilitating early disease detection and personalized medicine approaches. As the field advances, integrating oligonucleotide-based diagnostics with computational tools can enhance both throughput and precision in the identification of targets related to various illnesses.
Understanding the diverse applications of oligonucleotide secondary structures is crucial to unlocking their full potential in modern molecular biology and medical research.
Challenges in Studying Oligonucleotide Secondary Structures
Studying oligonucleotide secondary structures presents various challenges that can complicate both experimental and computational approaches. Understanding these challenges is crucial for anyone involved in research or application that relies on the proper functioning of oligonucleotides. Addressing these obstacles can lead to more robust findings and enhance the reliability of research outcomes.
Stability and Unfolding
One significant challenge in studying oligonucleotide secondary structures is their stability. Stability can be influenced by various factors, including temperature, ionic strength, and the identity of the nucleotide sequences themselves. For instance, factors like base pairing and related hydrogen bonds are critical in determining overall stability. As conditions change, oligonucleotides might undergo unfolding or conformational transitions, affecting their biological functionality.
Researchers often use specific techniques to assess stability. For example, fluorescence spectroscopy can quantify structural changes in real-time, revealing instability trends. However, there is no universal method for all sequences. Therefore, adaptations are necessary based on the specific oligonucleotide being studied.
Instability can lead to unpredictable experimental results. It can also hinder efforts in applications, such as gene editing or therapeutic development. Scientists must consider the conditions under which the oligonucleotides will operate to ensure accurate interpretations.
Experimental Variability
Another prevalent issue in the study of oligonucleotide secondary structures is experimental variability. Many factors in laboratory settings can introduce inconsistencies, such as variations in reagent quality, differences in measurement techniques, or even environmental conditions.
Considerations around oligonucleotide synthesis accuracy are paramount. Variability in synthesis can produce oligonucleotides with unintended modifications or impurities, complicating results. Different laboratories might use varying protocols, leading to discrepancies when comparing studies.
Reproducibility is crucial in scientific research. High levels of variability can undermine confidence in findings, making it difficult to validate results across studies.
To minimize these challenges, researchers can adopt standardized protocols and protocols. Additionally, employing advanced computational models to predict behavior under varying conditions can aid in reconciling some variability issues.
"The complexity of oligonucleotide behavior demands careful consideration of experimental parameters to achieve reliable results."
Future Directions in Oligonucleotide Research
Oligonucleotide research is an evolving field that significantly impacts genetic sciences and therapeutics. The future directions of this research hold immense promise, as they can influence our understanding of genetic mechanisms. By focusing on advancing technologies and interdisciplinary approaches, we can explore more effective solutions for genetic-related challenges.
Emerging technologies play a crucial role in continuing the evolution of oligonucleotide research. Advances in sequencing technologies, such as Next-Generation Sequencing (NGS), offer unparalleled insights into genetic variation. These methods facilitate the development of oligonucleotides that are finely tuned to target specific sequences and structures. Furthermore, CRISPR technology has revolutionized gene editing, enabling precise modifications at the genomic level. As these technologies advance, their integration with oligonucleotide design will likely lead to more effective therapeutic strategies and diagnostics.
Emerging Technologies
The introduction of various emerging technologies can reshape our understanding of oligonucleotide applications. Some key areas include:
- Nanotechnology: This field enhances oligonucleotide delivery systems. It enables the design of carriers that can promote targeted delivery of therapeutic oligonucleotides.
- Synthetic Biology: The concept allows researchers to construct new biological parts and systems. Scientists can design specialized oligonucleotides that can perform novel biological functions.
- Single-Cell Sequencing: It allows for the analysis of individual cells rather than bulk populations. Understanding the behavior of oligonucleotides within single cells can offer insights into variability and heterogeneity in biological systems.
The combination of these advances may pave the way for tailored treatments in precision medicine, treating genetic disorders more efficiently and with fewer off-target effects.
Interdisciplinary Approaches
The future of oligonucleotide research also relies on interdisciplinary approaches. Collaborations between fields such as bioinformatics, molecular biology, and computational sciences can yield new methodologies and applications.
- Bioinformatics: The integration of bioinformatics is essential for managing the vast data produced in oligonucleotide studies. Improved algorithms for analyzing sequence data will enhance prediction models for oligonucleotide behavior.
- Molecular Modeling: Techniques such as molecular dynamics simulations can elucidate how oligonucleotides interact with various cellular components. This knowledge can lead to better design principles for therapeutic applications.
- Chemistry Innovations: Research into novel chemical modifications can produce oligonucleotides with enhanced stability and bioavailability. These modified oligonucleotides may have improved efficacy in therapeutic contexts.
As we embrace these interdisciplinary strategies, the potential for innovative solutions in genetic therapies becomes more achievable. To harness their power, it is essential that researchers remain open to collaboration and exploration across various scientific domains.
"The future of oligonucleotide research is not just about the molecules themselves, but how they can be used in a broader biological context."
Closure
The conclusion serves as a critical synthesis of the insights presented on the topic of oligonucleotide secondary structures throughout this article. It reiterates the fundamental role these structures play in various biological processes, shaping our understanding of genetic functioning and therapeutic potential. Here, we recap the specific elements discussed, highlighting their interconnectedness and relevance.
Summary of Key Points
In summarizing the key points, we emphasize several critical aspects:
- Definition and Types: Oligonucleotides are short strands of nucleic acids essential in biotechnological applications. Understanding both DNA and RNA oligonucleotides is crucial in genetic engineering and therapeutic development.
- Importance of Secondary Structures: The formation of secondary structures—driven primarily by hydrogen bonding and base stacking—holds significant implications for their stability and functionality.
- Techniques for Analysis: Various cutting-edge techniques, including NMR spectroscopy and X-ray crystallography, facilitate the detailed study of these structures, ofer insights into their behavior in biological contexts.
- Applications and Challenges: Oligonucleotide secondary structures hold tremendous potential in gene regulation and as therapeutic agents. However, predicting and manipulating these structures present ongoing challenges which merit further exploration.
Significance in Current Research
The significance of oligonucleotide secondary structures in current research cannot be understated. They play a pivotal role in the advancement of genetic research and therapeutic strategies. By understanding these complex structures, researchers can improve the design of oligos for various applications, such as:
- Gene Editing: Secondary structures inform the design of CRISPR systems, enabling more precise targeting in genetic modifications.
- Therapeutics Development: Understanding the folding patterns aids in developing effective RNA-based therapeutics, such as RNA interference and antisense oligonucleotides.
- Molecular Diagnostics: Secondary structures facilitate the design of probes and primers used in diagnostic techniques, enhancing accuracy in detecting genetic mutations.
In summary, the exploration of oligonucleotide secondary structures not only enhances our fundamental biological understanding but also drives innovation in various fields, particularly in medicine and biotechnology. This domain continues to evolve, reinforcing the importance of ongoing research and interdisiplinary approaches.